James D. Muirhead1, Tobias P. Fischer2, Amani Laizer3, Sarah J. Oliva4, Emily J. Judd1, Hyunwoo Lee5, Emmanuel Kazimoto3, Gladys Kianji6, Cynthia J. Ebinger4, Zachary D. Sharp2, Josef Dufek7
1Syracuse University, 2University of New Mexico, 3University of Dar es Salaam, 4Tulane University, 5Seoul National University, 6University of Nairobi, 7University of Oregon
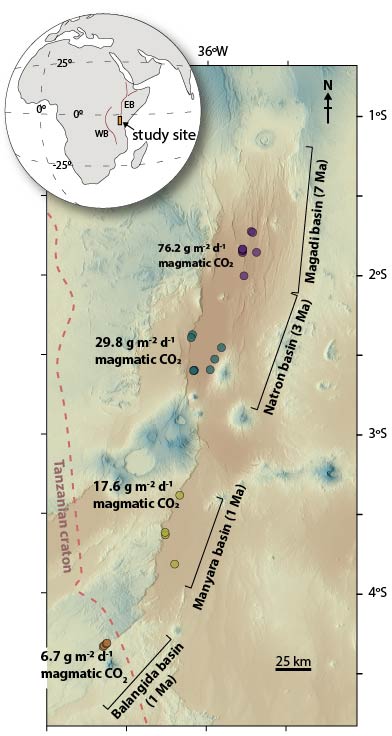
Figure 1. Annotated SRTM map showing the extent of the rift basins in the current study. Filled circles show the location of sampling regions within each basin, and the dashed brown line delineates the eastward-dipping surface boundary between the Tanzanian craton and Proterozoic mobile belt rocks (from the geological map of Thiéblemont et al. (2016)). Also included is the mean flux of magmatic CO2 from sampling sites in each basin. Inset in the top left shows the location of the DEM map on the African continent. Red lines show the extent of the Eastern (EB) and Western (WB) branches of the EARS.
In May and June 2018, our team of researchers completed the longest along-strike magmatic CO2 degassing survey in the East African Rift System (EARS) to date. Our CO2 flux data now extend over four rift basins, from the Magadi basin (Kenya) southward to the Balangida basin (Tanzania) (Fig. 1). During the 25-day field campaign, we collected over one thousand diffuse soil degassing flux measurements, and sampled hydrothermal spring systems along major fault zones to analyze the sources and fluxes of different volatile species. Here we present preliminary results of diffuse CO2 flux in zones within one hundred meters of observed spring discharge and use these values to examine variations in magmatic CO2 discharges between basins. The spatial variability of these data reveal how mantle CO2 fluxes in the EARS may evolve over the course of rift basin development, and are impacted by the initial composition and structure of the East African lithosphere.
Continental rifts are sites of lithospheric thinning and heating, which is commonly accompanied by magmatism and volatile transfer from Earth’s mantle to the lithosphere and atmosphere (White and McKenzie, 1989; Ebinger, 2005; Rooney, 2010; Lee et al., 2017; Foley and Fischer, 2017). They represent a key tectonic setting for natural CO2 emissions and possibly modulate Earth’s climate on geological timescales (Brune et al., 2017; Foley and Fischer, 2017). However, the total volume of mantle CO2 emitted at rift settings is poorly constrained, as are the mechanisms that control variations in CO2 flux over the lifetime of rifting.
The original carbon content of cratonic lithosphere is expected to be relatively low (~0.25 Mt C km–3 for 2-3 Ga lithosphere; Foley and Fischer, 2017). However, abundant carbon may be sequestered in the mantle lithosphere during the infiltration of both plume melts (e.g., Thompson et al., 2015) and carbon-rich hydrous-silicate melts generated during subduction (Foley and Fischer, 2017; Malusà et al., 2018).
These processes can potentially enrich carbon contents in the mantle lithosphere up to a hundred times above background values (Foley and Fischer, 2017). The resulting carbon accumulated during these events may be released during the generation and ascent of magma at continental rift settings (Malusà et al., 2018) (Fig. 2).
Although continental rifts represent potentially key sites of CO2 release, measuring the flux of CO2 from these settings is challenging and requires direct measurements and observations of CO2 discharge from zones of active rifting. The magma-rich Eastern branch of the East African Rift System (EARS) represents an ideal location to investigate these processes. Earlier degassing studies focused on direct measurements of volcanic plumes emitted from active volcanoes, such as Nyiragongo (Sawyer et al., 2008) and Oldoinyo Lengai (Brantley and Koepenick, 1995). In addition to these plume sources, EARS volcanoes release mantle volatiles to the atmosphere via springs, fumaroles, and zones of diffuse soil degassing, as well as during eruptive episodes (Darling et al., 1995; Fischer et al., 2009; Barry et al., 2013; de Moor et al., 2013; Hutchison et al., 2015; Lee et al., 2017). More recent studies in the EARS have shown that large volumes of mantle carbon are also released to the atmosphere along extensional fault systems situated away from volcanoes (Lee et al., 2016, 2017; Hunt et al., 2017). During this process, termed “tectonic degassing” (Burton et al., 2013; Lee et al., 2016), mantle carbon ascends to the surface along permeable fault zones and exits via springs, diffuse soil degassing zones, and gas vents (Muirhead et al., 2016; Lee et al., 2016, 2017; Hunt et al., 2017). This mantle carbon is primarily sourced from an enriched sub-continental lithospheric mantle and released into the crust and atmosphere by magmas emplaced at lower crustal depths (Lee et al., 2017; Roecker et al., 2017).
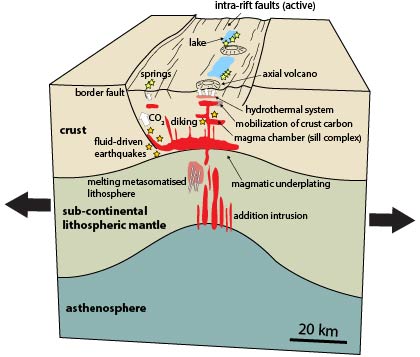
Figure 2. Production and transport of magmatic CO2 at continental rift settings modified from Hunt et al. (2017). White arrows represent zones of CO2 fluid flow, yellow stars are hydrothermal springs, and orange stars are deep earthquakes. The CO2 depicted exsolves from cooling upper and lower crustal magmas. The distribution of crustal magma (red polygons) is based on seismicity from Weinstein et al., (2017) and the seismic tomography model of Roecker et al. (2017).
Given the large aerial extent, pervasive faulting, and widespread magma emplacement occurring at depth in the EARS (e.g., Keranen et al., 2004; Roecker et al., 2017; Plasman et al., 2017), quantifying the volumes of CO2 released requires observations from a wide variety of structural settings along the rift system. Results of diffuse soil degassing surveys have thus far been reported from the northern and central Main Ethiopian Rift (Hunt et al., 2017) and Magadi-Natron basin (Lee et al., 2016), with estimates of 0.52-4.36 Mt yr-1 and 2.15-5.95 Mt yr-1 for each rift sector, respectively.
Extrapolation of these estimates point to potential CO2 fluxes on the order of 10-100 Mt yr-1, particularly when accounting for dissolved CO2 volumes transported in springs (Lee et al., 2017). However, these estimates do not consider the spatial and temporal variations of mantle CO2 discharge expected along any active rift system. The flux of CO2 within any rift basin should depend on a number of critical factors, such as the volume of carbon trapped within the underlying mantle lithosphere, rates of magma production, and the dissolved CO2 contents of ascending rift magmas (Foley and Fischer, 2017; Hunt et al., 2017). These variables are expected to vary both spatially and temporally within any continental rift setting, and quantifying their importance for mantle CO2 release requires extensive along-strike sampling of zones of volatile discharge.
Our recent field campaign was specifically designed to fill in these critical gaps in our understanding of rift CO2 fluxes, through an investigation of four segments of the Eastern branch of the EARS: the Magadi, Natron, Manyara, and Balangida basins (Fig. 1). These basins encompass a ~350 km-long stretch of continental rifting and range in age between 1 and 7 Ma, and are thus currently at different stages of development. Furthermore, these basins exhibit varying volcanic/magmatic fluxes and histories, and even cross the boundary between Proterozoic mobile belt rocks and the Archean Tanzania Craton (Fig. 1). Therefore, from these data we can assess:
- How mantle CO2 fluxes may evolve over the course of basin development; and
- How CO2 fluxes are impacted by the initial lithospheric composition and structure of the East African lithosphere.
Given the inherent variability of CO2 flux within individual rift basins (e.g., Hunt et al., 2017), when comparing CO2 discharges between basins it is critical to compare data from sites exhibiting similar structure, substrate, and hydrology. Therefore, we present here a subset of our collected data, focusing specifically on flux data (1) from rift-graben sediments, (2) in the vicinity of faults, and (3) in areas within 100 m of observed spring discharge.
The sources for diffuse soil CO2 discharges in volcano-tectonic settings are typically characterized as either biogenic or magmatic, with flux data in each population exhibiting a log-normal distribution and the highest mean flux observed in the magmatic population (e.g., Chiodini et al., 1998, 2008). Data from each study site, presented as probability plots in Figure 3, were sub-divided into two distinct populations by adapting the methodology of Sinclair (1974) into a newly designed MATLAB® code. This code iteratively fits biogenic and magmatic regression lines to the log-transformed data. Based on these functions, synthetic data sets are generated for each population and plotted against observed data, with the final solution being that which produces the highest R-squared and smallest root-mean-squared error values between the compared datasets. Outputs from this procedure provide an estimate of the percent contribution of biogenic and magmatic sources and their mean flux values.
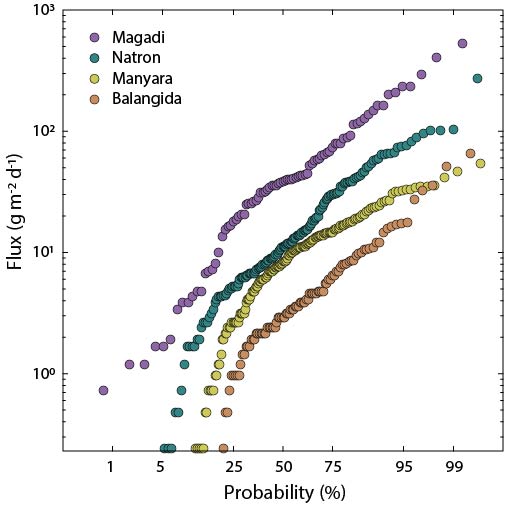
Figure 3. Probability plots of diffuse soil CO2 fluxes for each rift basin in the study. Note that the overall CO2 flux values decrease from north (Magadi) to south (Balangida). Flux values below the equipment detection limit (<0.24 g m-2 d-1) cannot be presented on the plots, but still affect the probability distribution of flux values above the detection limit.
Comparing data between basins, we observe a north to south decrease in both the percent contribution of the magmatic flux population and the mean magmatic flux value (see mean flux values in Figure 1). Lower magmatic CO2 flux values also correspond with younger rift basins (e.g., the Manyara and Balangida basins). These younger basins also exhibit lower volcanic/magmatic inputs (Le Gall et al., 2008; Albaric et al., 2014), which may relate to the low degree stretching and related decompression melting during this earlier stage of rifting, or to the relatively dry nature of thick Archean mantle that enables its preservation (e.g., Currie and van Wijk, 2016). Finally, as the locus of rifting gradually transitions from the Proterozoic mobile belt in the Natron basin, to the Tanzanian craton in the Balangida basin, we observe a significant reduction in the mean magmatic CO2 flux.
These preliminary results suggest that the volume of mantle CO2 discharge in the Eastern branch of the EARS is strongly dependent on the degree of lithospheric thinning, mantle hydration state, and related magmatism. The greatest mantle CO2 discharges in the EARS likely occur in more evolved systems outside the Archaean craton, such as the Kenya Rift (Lee et al., 2016) and Main Ethiopian Rift (Hunt et al., 2017). Furthermore, basins in their earliest rift stages (the ~1 Ma Manyara and Balangida basins) within Proterozoic mobile belt rocks exhibit higher CO2 fluxes than those in the Archean craton. This observation suggests that the Proterozoic lithosphere in East Africa may contain greater volumes of sequestered carbon, with its structure and composition suited for volumetrically significant CO2 discharges compared to the thick and probably dehydrated cratonic lithosphere. ■
Reference information