2016 ExTerra White Paper: Exploring Subduction through the Study of Exhumed Terranes
2016 ExTerra White Paper submitted to the Subduction Zone Observatory workshop
2014 ExTerra White Paper:
Understanding Convergent Margin Processes Through Studies of Exhumed Terranes
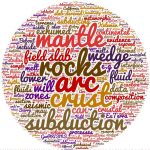
- Maureen Feineman (The Pennsylvania State University)
- Sarah Penniston-Dorland (University of Maryland)
- Jay Ague (Yale University)
- Olivier Bachmann (University of Washington)
- Ethan Baxter (Boston University)
- Gray Bebout (Lehigh University)
- George Bergantz (University of Washington)
- Susan DeBari (Western Washington University)
- Henry Dick (Woods Hole Oceanographic Institution)
- Mihai Ducea (University of Arizona)
- Oliver Jagoutz (Massachusetts Institute of Technology)
- Peter Kelemen (Lamont-Doherty Earth Observatory)
- Horst Marschall (Woods Hole Oceanographic Institution)
- Jeffrey Ryan (University of South Florida)
- Brian Savage (University of Rhode Island)
- John Shervais (Utah State University)
- Harold Stowell (University of Alabama)
Studies of rocks exhumed from paleo-subduction zones (Exhumed Terranes) contribute greatly to our understanding of the materials and processes that are hidden beneath the surface in active subduction zones. The goal of this white paper and of ExTerra is to organize research on exhumed terranes in order to accomplish more as a collective than we can as independent researchers. There are three target areas within active subduction zones that are subsequently exposed in exhumed terranes: middle and lower arc crust, the subducted slab, and the mantle wedge. In this document, key scientific questions are identified for each target area along with a summary of relevant recent scientific progress for each question. Interdisciplinary field institutes are proposed as a primary venue for collective research on exhumed terranes. In these field institutes scientists will travel together to map, discuss, and collect samples. The institutes will generate collaborative research, with published results that will enhance understanding of active subduction zone processes within the broader community. Together, researchers will explore specific science questions identified by this white paper. Examples of proposed field institutes and associated science questions are outlined herein. Finally, a plan for data and sample management using existing systems such as the International GeoSample Number (IGSN) sample registration and MetPetDB database is proposed.
The National Science Foundation GeoPRISMS Subduction Cycles and Deformation (SCD) Science Plan identified the study of exhumed terranes as an important component of subduction zone research. During active subduction, rocks of the subducting slab, mantle wedge, and arc crust are located deep beneath the Earth’s surface. As a result, direct observation of these rocks can only be carried out subsequent to exhumation. The nature of exhumation processes is such that entire subduction zones are rarely, if ever, exposed in a single location. Therefore, studies of these rocks are conducted at multiple locations representing different paleo-subduction zones most often by multiple research groups, using different techniques and approaches, with the goal of representing a comprehensive range of structural settings and slab pressure and temperature conditions. Currently, the study of exhumed terranes is included in the GeoPRISMS implementation plan as a thematic approach. The goal of this white paper is to explore how we can best organize research on exhumed terranes to accomplish more as a collective than we could as individuals working independently.
The GeoPRISMS SCD Science Plan identifies seven key questions to be addressed by research covered under this initiative. Studies of exhumed terranes contribute directly to at least five of these seven key questions, including:
4.2 How does deformation across the subduction plate boundary evolve in space and time, through the seismic cycle and beyond?
4.3 How do volatile release and transfer affect the rheology and dynamics of the plate interface, from the incoming plate and trench through to the arc and backarc?
4.4 How are volatiles, fluids, and melts stored, transferred, and released through the subduction system?
4.5 What are the geochemical products of subduction zones, from mantle geochemical reservoirs to the architecture of arc lithosphere, and how do these influence the formation of new continental crust?
4.6 What are the physical and chemical conditions that control subduction zone initiation and the development of mature arc systems?
There are many places on Earth where exhumed rocks from subduction zones can be directly observed in the field. Metamorphic and igneous geologists have the unique ability to inform studies of active subduction zones by providing “ground-truth” verification of the assumptions that go into models, experiments, and interpretive geophysics and geochemistry. Some of the contributions ExTerra aims to provide to the GeoPRISMS community include:
- Linkage between experiments / models / seismic observation and physical reality: (1) Concrete evidence of processes progressing through space and time, (2) Verification of scaling factors – we can compare grain scale to outcrop scale, outcrop scale to seismically resolvable features, and days in the lab to thousands or even millions of years in the field
- Organization of individual efforts into major interdisciplinary objectives, wherein the whole is greater than the sum of the individual contributions
- Integration of data from diverse localities, allowing coverage of a broad range of conditions not observable at a single focus site
- Coupled study of the products of mechanical and chemical processes in situ
- Fine-tuning of sample and data collection to better serve the needs of other groups (for example, we envision geochemists helping seismologists, petrologists helping modelers, etc.)
The objective of this white paper is to demonstrate the need to make the study of exhumed terranes an integral part of the GeoPRISMS SCD Initiative. Three target areas have been identified as significant to improving our understanding of active subduction processes and accessible through study of exhumed terranes:
• Middle and lower arc crust: Exhumed rocks include granitoids, gabbros, metasedimentary rocks (commonly migmatized), gneisses, amphibolites, pyroxenites (with or without garnet), and granulites.
• Subducted slab: Exhumed rocks include high-pressure (HP) and ultrahigh-pressure (UHP) rocks such as blueschists, eclogites, and metasedimentary rocks.
• Mantle wedge: Exhumed rocks include serpentinites, ophiolites, peridotites, and variants thereof (dunites, pyroxenites, etc.).
Studies of exhumed middle and lower arc crust can provide key spatial and temporal constraints on the evolution of arc magmas that cannot be addressed directly through studies of lavas erupted at the surface. Such studies will allow for assessment of the temporal evolution of subduction-related magmatism from initiation until collision and beyond, providing a complex integrated picture of continental crust formation and evolution over time. Additionally, studies of arc crust will allow for field verification of models that have been developed through geochemical and geophysical studies of active subduction zones by allowing us to directly observe features that may support or refute geological paradigms. For example, this may include evidence for continental crustal evolution through the process of lithospheric delamination (e.g. Kay et al., 1994), the existence of a “lower crustal hot zone” (Annen et al., 2006), or the cyclical thickening and thinning of the upper plate lithosphere in subduction systems (DeCelles et al., 2009).
Field observations made along high-resolution crustal profiles, coupled with petrologic, geochemical, and geophysical measurements, will provide an integrated thermal, compositional, and mechanical dataset that will allow for the generation of representative seismic velocity profiles for middle and lower crust. This will facilitate the interpretation of seismic velocity data and allow for better correlation of seismic data with the structure and composition of middle and lower crust.
Some of the key scientific questions to be addressed by the study of arc crust include:
- What are the geochemical products of subduction that influence formation and evolution of continental crust (4.5)?
The question of how continental crust forms and evolves is still a matter of debate, but the answer almost certainly includes a significant contribution from subduction zones. Theories for establishment of the oldest continental cratons, as represented by Archean trondjhemite-tonalite-granodiorite (TTG) series, include partial melting of basalts in hot, early subduction zones (Rapp et al., 2003), and modern analogs are believed to form by partial melting of underplated basaltic materials at the base of arc crust (Atherton and Petford, 1993; Jackson et al., 2005; Kidder et al., 2003). Both the lavas that erupt at volcanic arcs and the plutons that accumulate below the surface are hypothesized to represent the primary products that led to the long-term growth and evolution of the crust.
- What are the fluxes into and out of arc crust over time (4.4, 4.5)?
Mass fluxes into arc crust include mantle- and perhaps slab-derived melts with contributions from solute-bearing fluids from the subducting slab. Mass fluxes out of arcs include lower crustal delamination and weathering and erosion of materials entering rivers and oceans (Rudnick, 1995; Lee et al., 2008). Much of the evidence for lower crustal delamination is theoretical or indirect (Zegers and van Keken, 2001; Willner et al., 2002; Dufek and Bergantz, 2005; Massonne, 2005). The process is inferred from observations indicating changes in the thermal state and composition of lithospheric sections through time (e.g. Ducea and Saleeby, 1996, Lee et al., 2001), as determined by xenolith studies. It is also inferred from thermal and geochemical changes in surface magmatism (e.g., Kay et al., 1994; Ducea et al., 2013). Geophysical evidence also suggests that recently detached Raleigh Taylor instabilities from beneath arcs exist (e.g. Zandt et al., 2004 for the southern Sierra Nevada area, California). In addition, there is clear field evidence for this process in unique instances where the island arc paleo-Moho is exposed in exhumed terranes (Greene et al., 2006; DeBari and Greene, 2011; Jagoutz et al., 2011; Jagoutz and Schmidt, 2012). Although it may be difficult to identify clear physical evidence for a process of which the primary physical manifestation is an absence of material, it is logical that catastrophic lithospheric delamination will bring fresh, hot mantle materials into contact with the remnant lower crust. Also following delamination, isostatic rebound is expected due to the loss of dense crustal roots (e.g., Levander et al., 2011). Thus petrologic evidence for rapid heating and exhumation of lower crustal rocks may provide supporting evidence for delamination (Willner et al., 2000, 2002; Massonne, 2006). Recent work also suggests that relamination of the lower crust by partial melts of subducted sedimentary rocks may result in more felsic lower crust than previously believed (Hacker et al., 2011).
- What is the extent of exchange between arc magmas and arc crust? How do cumulates, restites, and wall rocks influence magma evolution (4.4, 4.5)?
Arc magmas are believed to evolve within the crust through processes of fractional crystallization and assimilation of or hybridization by partial melts of the crust (DePaolo, 1981; Hildreth and Moorbath, 1988; Dufek and Bergantz, 2005; Annen et al., 2006). The significance of these processes is inferred from magma compositions, but somewhere within the middle and lower crust there should exist direct evidence in the form of cumulates formed during fractional crystallization and/or restites resulting from melt extraction. For example, cryptic fractionation of amphibole and/or garnet is believed to control the rare earth element trends in many arc magmas (Davidson et al., 2007, Macpherson et al., 2006; Coldwell et al., 2011; Girardi et al., 2012). In fact, the great majority of continental arc magmas worldwide have rare earth element (REE) signatures consistent with fractionation or partial melting from a deep, garnet-rich and plagioclase-poor hot zone (Gromet and Silver, 1987; Girardi et al., 2012). In-place hornblende- and garnet-bearing cumulates have been directly observed in the Talkeetna Arc and Kohistan Arc crustal cross-sections, and correspond to magmatic evolution trends in these arcs (Greene et al., 2006; Jagoutz, 2010), and are also observed as deep lithospheric xenoliths, best known from the Sierra Nevada arc in California (Ducea and Saleeby, 1998; Lee et al., 2001; Chin et al., 2012).
Exhumed deep arc roots of Cordillera-style batholiths in the Americas are relatively few. Among the best known are (1) the Ordovician Sierra Valle Fertil-La Huerta in Argentina, an exposed section though the Famatinian arc (Otamendi et al., 2009; Ducea et al., 2010), (2) the Mesozoic Coast Mountains Batholith Complex in NW Washington State (Miller et al. 2009a, 2009b; Dessimoz et al. 2012) and British Columbia/SE Alaska (Gehrels et al., 2009; Girardi et al., 2012), (3) various dismembered pieces of the southern California “allochthon”, such as the Salinian block, the San Emigdios, and parts of the San Gabriel Mountains in California (Kidder et al., 2003, Chapman et al., 2012; Barth et al., 1995) and (4) the southern Sierra Nevada tilted exposure (Pickett and Saleeby, 1993). All of these exposures contain relatively (structurally) intact sections that have been tilted and exposed to crustal levels as deep as 35 km. The Ordovician Famatinian Arc, exhumed in western Argentina, provides a cross section of a mid-crustal magma chamber frozen in the act of assimilating partially melted crustal rocks (Otamendi et al., 2009, 2012; Ducea et al., 2010). This clearly indicates that arc magma compositions are developed through complex processes occurring in the lower arc crust that depend on a number of factors such as lithological variability, thermal conditions, fO2, and H2O content of the melt. Only through the careful studies of numerous exhumed terranes such as these can we understand the control of specific parameters on crustal evolution and ultimately use this information to better constrain numerical models (e.g., those of Dufek and Bergantz, 2005, or Annen et al., 2006).
- How and where is magma transported and stored in the crust (4.4)?
It is generally accepted that the continental crust (broadly speaking) and at least some arc crust is chemically stratified into a more felsic upper crust (see below) and a more mafic lower crust. How and where this chemical stratification occurs is poorly understood. Related to this observation is the question of how melts are transported and stored in the arc crust. For example, it has been postulated that a line of neutral buoyancy exits in arcs where melts are denser than surrounding rocks and accordingly stagnate and differentiate. The existence of such a buoyancy line however, has rarely been documented through field studies. For example, this transition is observable in the Sierra Valle Fertil in the Famatinian arc (Otamendi et al., 2009) and in theory should be easy to detect elsewhere. Similarly, it is debated whether melts are dominantly transported through the crust by elastic dyke propagation or as massive, positively buoyant diapirs; the answer should be observable in exhumed arc crust.
- How variable is the bulk composition, fabric, melt/fluid content, and thermal structure of arc crust, and how might these properties influence our interpretation of seismic velocity profiles (4.5)?
Interpretation of seismic velocity data requires some knowledge of the physical properties of the mantle and crustal materials through which seismic waves propagate. For example, recent seismic studies have identified a 2-8 km thick zone of P-wave velocities of 6.0-6.5 km/s in the middle crust at many island arcs (e.g., Izu-Bonin-Mariana (IBM): Takahashi et al., 1998, 2007; Kodaira et al., 2007; Calvert et al., 2008), and experiments suggest that this zone is consistent with a felsic-plutonic middle crust (Kitamura et al., 2003). The accreted Talkeetna Arc in Alaska and Kohistan Arc in Pakistan provide complete cross-sections through the roots of comparable island arcs, in which the exposed middle crust can be observed directly. Both of these cross-sections contain an intermediate to felsic plutonic complex in the middle crust (Rioux et al., 2010; Jagoutz et al., 2010; Jagoutz and Behn, 2013), supporting the theoretical and experimental interpretations of the seismic data observed in the IBM and many active arcs (except the Aleutians). There are numerous batholiths that expose examples of what this low velocity middle crust may look like (Sierra Nevada, Baja, Coast Plutonic Complex, Fjordland, etc.). In addition, bulk composition, mineralogy and deformation fabric (preferred orientation) all contribute to the seismic properties of middle and lower crust, making interpretation of seismic velocity profiles a complex and potentially rewarding undertaking (Barruol and Kern, 1996; Tatham et al., 2008; Lloyd et al., 2009, 2011). Studies of exhumed terranes provide an excellent opportunity to directly observe the stratified petrology and deformational features that contribute to the vertical complexity of the middle and lower crust. Drilling to the middle crust in an active arc has also been proposed as a way to directly sample lithologies with these P-wave velocity attributes (i.e., drilling into the mid crust of the IBM arc with Chikyu; http://www.jamstec.go.jp/ud2012/)
- To what extent are the volcanic products of subduction zones representative of the hidden plutonic components in the crust? What percentage of arc magmas is erupted at the surface, relative to what is retained in plutons (4.4, 4.5)?
Textural observations and geochemical-mineralogical evidence in both plutons and volcanic units suggest that plutonic products are in some instances frozen magmatic products, whereas in others crystal-rich residues that complement the volcanic output. These relationships are particularly well documented in mafic systems (e.g., Sinton and Detrick, 1992; Greene et al., 2006; Humphreys, 2009; Jagoutz, 2010; Vantongeren et al., 2010) but are also noticeable in intermediate to silicic compositions in many locations around the world (Bachl et al., 2001; Deering and Bachmann, 2010; Deering et al., 2011; Beane and Wiebe, 2012). The percentage of arc magmas erupted at the surface, relative to what is retained in plutons, can be estimated using physical models of crystal-liquid separation coupled with volume assessments of volcanic deposits (e.g., Dufek and Bachmann, 2010; Gutierrez and Parada, 2010; Deering et al., 2011). This problem can also be approached by observation of exhumed crustal cross-sections. At the Talkeetna Arc, where the complete volcanic section is exposed and accounts for 7 km of the ~35 km-thick crustal section (Hacker et al., 2008), calculated liquids in equilibrium with clinopyroxene in the lower crustal cumulate section (gabbronorites) are an exact match for the volcanics preserved in the upper crust, suggesting that the volcanics and cumulates evolved from the same parent magma (Greene et al., 2006). Cordilleran arc exposures can also be used to determine the percentage of intrusive to extrusive igneous crust, assuming that a sedimentary record documenting the mass of eroded stratovolcanoes exists – this is the case for defunct, but relatively young, North American arcs such as the Sierra Nevada. Merging models of multi-phase fluid dynamics in magma reservoirs with direct observations from the volcanic and plutonic record is an important direction to further constrain mechanisms of crust formation.
- What is the mode of emplacement of metasedimentary rocks in arc lower crust (~1 GPa)? Are they gradually buried, thrust laterally into the crust, or diapirically emplaced from below (4.5)?
Metasedimentary rocks appear to be relatively common in the lower crust in a number of continental margin arcs, based on recently documented examples from the North American cordillera (e.g., Whitney, 1992; Whitney et al., 1999; Kelemen et al., 2003; Matzel et al., 2008; Evans and Berti, 1986; Brown and Walker, 1993; Pickett and Saleeby, 1993; Saleeby et al., 2003; Ducea et al., 2009). In fact, it is quite rare to see older basement rocks in deep crustal sections of continental arcs – the only rocks other than the newly added mantle-derived melts and derivative igneous rocks are meta-sedimentary sequences that equilibrated at HP to UHP conditions and commonly show evidence of migmatism. Though metasedimentary lithologies are uncommon in the oceanic Talkeetna and Kohistan arc sections, there is evidence for supracrustal, volcanic rocks in the lower crust in such settings (Kelemen et al., 2004; 2014). A variety of processes have been invoked to explain the presence of surficial rocks in the lower crust. Specific explanations for the North American examples include (1) gradual burial and envelopment in the growing arc edifice, (2) vertical, downward flow in response to corresponding upward emplacement of (hot, felsic, buoyant) plutonic rocks, (3) underplating of metasedimentary rocks from the subducting plate onto the lower arc crust, in the absence of an intervening mantle wedge, and (4) tectonic thickening of the section via subduction erosion and/or imbrication of fore-arc and back-arc basin sequences into arc lower crust. More generally, several recent studies have focused on the fate of subducting, buoyant, subducted metasedimentary and felsic igneous rocks, and of buoyant continental crust in collision zones (Behn et al., 2011; Currie et al., 2007; Hacker et al., 2011; Whitney et al., 2009). Return of these buoyant materials via flow up a subducton channel and/or via diapirs ascending through the mantle wedge, termed “relamination” by Hacker et al. (2011), seems likely on geological and theoretical grounds.
Productive future research could include development and use of methods for distinguishing between different proposed mechanisms for formation of continental lower crust, which mainly involve subduction processes: (1) delamination of dense lithologies from the base of arc crust, and (2) relamination of buoyant lithologies rising from subduction zones. In hypothesis (1), continental lower crust should be similar to arc lower crust after subtraction of dense lithologies that may be present at the crust-mantle boundary, such as garnet granulites and ultramafic plutonic rocks (e.g., Arndt and Goldstein, 1989; Herzberg et al., 1983; Jull and Kelemen, 2001; Kay and Kay, 1991; Ringwood and Green, 1966). In hypothesis (2), continental lower crust should be similar to buoyant lithologies in arc crust, particularly arc upper crust.
- What is the bulk composition of continental arc crust? What is the bulk composition of oceanic arc crust? How and why do they differ (4.5)?
Detailed studies of oceanic arc crustal sections have led to a number of recent summaries and reviews (e.g., DeBari and Greene, 2011; Jagoutz and Schmidt, 2012; Kelemen et al., 2004; Kelemen et al., 2014). There are two nearly complete, tectonically exposed sections of arc crust, the Jurassic Talkeetna arc in south central Alaska, and the Cretaceous Kohistan arc in northwestern Pakistan. Thermobarometric data suggest that there may be a structural gap in the Talkeetna arc section, and as a result the bulk composition of the arc is uncertain, ranging from basaltic (DeBari and Sleep, 1991; Greene et al., 2006), to andesitic (Behn and Kelemen, 2006; Hacker et al., 2008). For the tectonically intact Kohistan arc, incorporating thermobarometric and geochemical data, Jagoutz and Schmidt (2012) determined an andesitic bulk composition, in contrast to previous basaltic estimates (e.g., Miller and Christensen, 1994). The Talkeetna section preserves a narrow horizon – a few hundred meters thick – of garnet granulites and pyroxenites between predominant, lower crustal gabbronorites and residual upper mantle harzburgites. It has been inferred that the garnet granulites and pyroxenites represent a much larger mass of such dense lithologies, which foundered into less dense, underlying mantle peridotites. In Kohistan, by contrast, a relatively thick layer (~10 km) of garnet granulites and ultramafic cumulates remains at the base of the section.
The bulk composition of the continental arcs is less well known. In general, the bulk composition is believed to lie between andesite and dacite in the volcanic cover and the upper 20-30 km of arc crust (Ducea, 2001; 2002). After a transition to a denser arc root, granulite facies rocks extend to about 45 km (Ducea et al., 2003), and are followed at greater depths by dense pyroxenites that may be prone to recycling (Saleeby et al., 2003). Major element similarities between the Kohistan arc section, possible andesitic compositions for the Talkeetna arc, and estimated, bulk continental crust have led to proposals that these arc sections – after “delamination” of dense lithologies – represent juvenile continental crust. However, further testing of this hypothesis is needed, particularly via comparisons of the remaining, “density stable” arc lower crust with granulite terrains and xenoliths representative of continental lower crust, as outlined in the previous section.
Studies of exhumed rocks from the subducted slab and its overlying sediment section address key questions involving the role of volatiles, fluids and melts, geochemical cycling, the nature of the slab-mantle interface, and the end-products of metamorphic processes that are returned to the deep mantle after processing within subduction zones (see Bebout, 2014). Investigation of exposed high-pressure and ultrahigh-pressure terranes will allow disentanglement of processes of mixing and material transport occurring within the subducting slab. Interpretation of isotopic and elemental signals in arc volcanic rocks, as well as of geophysical data collected from active subduction zones, relies ultimately on understanding processes of fluid generation and transport within and above the subducting slab.
Field observations made along with petrologic, geochemical, and geophysical measurements on samples of HP-UHP rocks and associated mélange terranes can provide thermal, compositional, and mechanical data useful in constraining the seismic velocity characteristics of these materials. This will facilitate the interpretation of seismic velocity data and allow for better correlation of seismic data with the structure and composition of the subducted slab and the slab-mantle wedge interface. Petrologic, thermodynamic, and geochronological analysis of exhumed HP-UHP rocks also provide a field-based test for geodynamic model predictions of subduction zone thermal structure and evolution as well as the spatial and temporal scales of devolatilization.
Some of the key scientific questions to be addressed by the study of exhumed rocks from the subducted slab include:
- What is the composition of slab-derived fluids and melts and how do these compositions change with depth (4.4)?
Much debate has surrounded the physical state and chemical composition of the mobile component released from the slab along the prograde path. As pressure and temperature increase along the subduction prograde path, aqueous fluids, silicate melts, and/or supercritical fluids may be released. Information about the composition of slab-derived fluids is largely derived indirectly from elemental variations in arc lavas (Stolper and Newman, 1994; Class et al., 2000), experimental investigations (Schneider and Eggler, 1986; Brenan et al., 1995; Kessel et al., 2005), or theoretical calculations (Manning, 1998). Exhumed HP-UHP rocks can retain remnants of melts and/or fluids in the form of leucosomes, veins, or fluid inclusions in minerals, providing a direct record of element mobility in the slab (Sorensen and Barton, 1987; Bebout, 2012). For example, the observation of rutile in veins and fluid inclusions in eclogite tells us that, under some conditions, TiO2 can be soluble in slab fluids (Gao et al., 2007; Philippot and Selverstone, 1991) – a result that would not be arrived at on the basis of arc lava compositions, which are characteristically depleted in TiO2 and other high field strength elements. Studies of diamond-bearing fluid inclusions in exhumed UHP rocks can also help to elucidate the role of subduction in Earth’s deep carbon cycle (Frezzotti et al., 2011). More mineral-scale data from natural rocks are needed to complement experiments constraining the solubility of minerals and partitioning of elements between minerals and fluids. Recent developments in the analysis of fluid inclusions (Allan et al., 2005; Scambelluri and Philipot, 2001) should allow more information to be obtained directly from microscopic samples of slab-derived fluids using laser ablation. Additionally, information from rocks of the subducted slab, especially from the stable isotopic composition of the rocks, can help constrain the lithologic source of fluids (e.g. Bebout and Barton, 1989; Sadofsky and Bebout, 2004; Bebout et al., 2013a, b).
- What are the pathways, fluxes and timescales of fluid release in the slab (4.4)?
Models of fluid release based on thermodynamic equilibrium (e.g., Hacker et al., 2003; Hacker, 2008; van Keken et al., 2011; Baxter and Caddick, 2013) predict volumes of fluid retained or released through the process of subduction. There is evidence for fluid flow in the form of veins and altered rocks in mélange terrains in subduction zone metamorphic rocks (Bebout, 1991; Breeding and Ague, 2002). Investigation of these features provides insight into mechanisms of fluid transport and fluid-flow paths within subduction zones. Constraining the flux of fluids through subducting slabs is difficult without proper spatial constraints, but can provide an important test of model predictions. For example, analysis of crosscutting veins and pseudotachylytes in eclogites has led to the interpretation that hydraulic fracturing and channelized fluid flow in the slab are initiated by intermediate-depth earthquakes (John and Schenk, 2006). Recent efforts with geospeedometers suggest rapid timescales of fluid-rock interaction in subduction-related metamorphic rocks on the order of hundreds of years (e.g. Penniston-Dorland et al., 2010; John et al., 2012). Geochronology of dehydration related to garnet growth also suggests short bursts of fluid production from subducting lithologies (e.g. Dragovic et al. 2012).
- What is the detailed thermal evolution of the slab (4.2, 4.4)?
The distribution of elements in major phases in metamorphic rocks provides data regarding the temperatures and pressures of equilibration of those phases, assuming equilibrium. Mineral inclusions within garnets can additionally constrain relative timing that enables us to construct generalized pressure-temperature-time (P-T-t) paths (King et al., 2004). When the minerals can be radiometrically dated, we can arrive at a detailed thermal evolution of the slab through time, and recent developments in high-resolution isotopic analysis are allowing researchers to date zones within the garnets themselves (Pollington and Baxter, 2010, 2011; Dragovic et al., 2012). However, some minerals are exceedingly difficult to date, in particular many stabilized at blueschist facies conditions. Nonetheless, progress has been made in recent years, for example in the development of new techniques to date lawsonite (Mulcahy et al., 2009) and in situ techniques for dating titanite and rutile (Zack et al., 2011; Spencer et al., 2013). Petrologically-derived P-T-t paths provide much-needed constraints for thermal models of subduction zones, and can be used to test the predictions of these models. These models in turn will help with the interpretation of seismic models, as both viscosity and wave speed are significantly affected by temperature.
- What are the reaction and diffusion rates at conditions relevant to subducting slabs? To what extent do metamorphic reactions “keep up” with changing pressure-temperature conditions in the slab (4.4)?
The above arguments regarding thermobarometry and geochronology rely upon the assumption of thermodynamic equilibrium among minerals. Moreover, models that predict the depths and amounts of fluid release from the slab also rely upon the same assumption of equilibrium. How reasonable is this assumption at low temperatures such as those found in slab-derived blueschists and eclogites? Field measurements of metamorphic reaction rates, combined with experimental determination of diffusion rates in minerals and reaction rates in rocks, are enabling us to develop generalized rate laws in order to quantitatively address this question (Baxter, 2003; Cruz-Uribe et al., 2014). Existing data suggest that known regional reaction rates are too slow to maintain equilibrium in cold, rapidly subducting slabs such as North Honshu (Cruz-Uribe et al., 2014). Calling upon fluids and/or rapidly changing thermal conditions as catalysts for increasing reaction rates may be necessary in order to meet the constraint of significant dehydration of the slab prior to or upon reaching sub-arc depths.
- What is the nature and composition of the rocks at the interface between the downgoing subducting slab and the overlying mantle wedge and how do they change with depth (4.2)?
Geophysical imaging supports the existence of 2-8 km thick low-velocity layers on top of subducting slabs (Abers, 2005; Kim et al., 2013) and confirms the presence of a cold “nose” of serpentinized mantle wedge in the forearc region (Bostock et al., 2002; Abers et al., 2006). Yet, in detail, the nature and structure of the subduction interface are still poorly constrained. Exposures of rocks representing this interface are of two major types: large scale (>km) slices with relatively coherent P-T estimates (e.g. Western Alps, Angiboust et al., 2009) and mélange zones consisting of isolated fragments of eclogite and blueschist in serpentinite- or mud-rich matrix (e.g. Franciscan Complex) with petrological hints of punctuated exhumation and reburial (Wakabayashi, 2012). This latter type (Sorensen and Barton, 1987; Grove et al., 2008), tends to record more extensive fluid flow (Bebout and Barton, 1989; Sadofsky and Bebout, 2004), and suggest a flowing, active subduction channel (Cloos, 1982), whereas the larger tectonic slices from the former type record colder, less dynamic, less fluid-rich conditions (Philippot and Selverstone, 1991; Agard et al., 2009). It is uncertain whether the larger “coherent” expanses of HP/UHP rocks simply represent larger slices or slabs in similarly structurally disrupted zones (i.e., as at the Monviso locality, Italian Alps). In order to properly interpret the results of geophysical imaging, these rocks must be fully characterized, including information about the mineralogical, chemical, rheological and structural characteristics of the varying lithologies along with determination of the abundances of these lithologies within the different types of plate interfaces. Mixed rocks such as are commonly found in mélange zones (e.g. Bebout and Barton, 2002; Marschall and Schumacher, 2012; Penniston-Dorland et al., 2012a) add complexity beyond what can be predicted from the comparison of subduction factory “inputs” to subduction factory “outputs” (see also King et al., 2006, 2007; Spandler et al., 2008).
- How is the slab signature transferred to the mantle? (4.3)?
It has been generally accepted that likely mechanisms for transfer of a slab-derived geochemical imprint to the sub-arc mantle wedge include mobilization of elements from the slab by aqueous fluids generated by dehydration reactions at increasing pressure and temperature (e.g., Ellam and Hawkesworth, 1988; McCulloch and Gamble, 1991) and/or by partial melts generated within the subducting slab (e.g. Elliott et al., 1997). However, there is increasing evidence that mechanical processes such as mélange flow, diapirism, underplating, and/or imbrication of the slab may contribute to the slab-derived signature. Geodynamic modeling of subduction zones and comparison of metamorphic rocks to arc volcanic rocks suggest that buoyancy-driven diapirs may transport slab material into the hotter mantle wedge, at which point melting may occur (Gerya and Yuen, 2003; Behn et al., 2011; Marschall and Schumacher, 2012). Evidence from UHP meta-sedimentary rocks, including those exposed in the Erzgebirge, Germany, suggests that some characteristic trace element signatures thought to be associated with partial melting of the slab may be retained until much higher temperatures than are typically modeled at the surface of the subducting slab at depths of arc magma generation (Behn et al., 2011). Evidence from rocks of the Catalina Schist suggests that both physical mixing of materials derived from subducted slab and mantle wedge (Bebout and Barton, 2002; Penniston-Dorland et al., 2012a) and transport of elements by fluids and melts occur within the melange zone (Sorensen and Barton, 1987; Bebout and Barton, 1989; Sorensen and Grossman, 1989; Bebout, 1991; 1997; Penniston-Dorland et al., 2012b). These processes could potentially create the mixed signal observed in chemical signatures at the slab-mantle interface that is then transported upwards through diapiric rise (Marschall and Schumacher, 2012). Further petrologic, geochemical and structural studies of these rocks and the differences among them can reveal whether this is a plausible mechanism for generating partial melting underneath arc volcanoes.
- What can the rock record reveal about mechanical behavior associated with subduction-related seismic events (4.2)?
Pseudotachylytes in eclogite from Zambia (John and Schenk, 2006) and eclogite breccia in the Monviso ophiolite (Angiboust et al., 2012) provide direct evidence for brittle behavior of rocks at depths >65 km and a physical record of earthquakes in the sub-arc region of a (previously) active subduction zone. Observation and analysis of these rocks enables us to determine how the properties of subduction-related rocks affect the nature of the slab-mantle interface and the range of brittle processes including everything from megathrust earthquakes to episodic slip and tremor.
- What happens at the 80 km depth coupling boundary (4.2)?
A critical component of subduction zones is the nature of coupling between the downgoing subducting slab and the overriding mangle wedge. Low surface heat flow in the forearc contrasts with high heat flow in sub-arc and back-arc settings. This thermal difference is thought to be controlled by the onset of mantle wedge flow beneath the arc and its absence in the forearc. Absence of mantle flow in the forearc requires that the mantle wedge be decoupled from the subducting slab. Numerical thermal models predict decoupling of slab and mantle to depths of 70-80 km (e.g. Wada and Wang, 2009) and recent geochronology and thermodynamic modeling of blueschist shows a sharp thermal gradient near this depth (Dragovic et al. 2012), consistent with these predictions. Subduction-related metamorphic rocks are exhumed from these depths, and the nature of the coupling interface can potentially be investigated through study of these rocks.
Studies of mantle rocks inform modelers and geophysicists by providing constraints on the thermal and seismic properties of the mantle wedge. Field observations allow us to bridge the scaling gap between very small experimental assemblies (a few cubic centimeters at best) and global-scale mantle dynamics. The fabric and orientation of mantle rocks provides permanent and direct indicators of mantle rheology and deformation across the slab/mantle boundary. The mineralogy and petrology of mantle rocks provides information regarding melt formation and transport, and also regarding the enrichment of the mantle wedge by interaction with slab-derived fluids and melts (mantle metasomatism).
It is widely recognized that serpentinization of the mantle is likely to play a significant role in a range of first-order problems, including controlling arc fluid compositions, volatile budgets, seismic wave velocities, and dynamics in the “nose” of the mantle wedge. In addition, carbonation accompanying serpentinization of peridotite and subduction of ophicarbonates (i.e., carbonated serpentinites and peridotites) may be a significant global carbon sink.
Some of the key scientific questions to be addressed by the study of exhumed rocks from the mantle wedge include:
- How does the addition of fluid or melt from the slab affect the composition of the mantle wedge (4.5)?
It is generally accepted that the addition of slab-derived fluids to the mantle wedge alters the composition of the mantle by enriching it in fluid mobile elements, contributing U-series nuclides in secular disequilibrium, and oxidizing the wedge (McCulloch and Gamble, 1991; Elliott et al., 1997; Turner et al., 1997; Blatter and Carmichael, 1998; Feineman et al., 2007; Kelley and Cottrell, 2009; Shervais and Jean, 2012). Most of these interpretations are inferred from end-product arc basalt compositions. With the exception of U-series disequilibrium, which is too short-lived to be recorded in exhumed rocks, these interpretations beg for confirmation by direct observation in mantle rocks. If the overall mantle wedge composition, including the fluid and melt contents, are poorly known, then making interpretations from geophysical observables will be problematic. Field observation and sampling of subduction-altered mantle materials will allow us to verify and quantify the effects of slab-derived fluid addition on mantle wedge composition, and further constraints on the amount of melt and how it is stored in the wedge (in minerals, in pore space, etc.) will greatly improve the accuracy of geophysical interpretations.
- How does the addition of fluid affect the rheology of the mantle wedge (4.3)?
It has been shown experimentally that addition of water to mantle minerals weakens them, inducing changes in mantle rheology and seismic wave attenuation (Hirth and Kohlstedt, 1996, 2003; Karato and Jung, 1998). This same affect induces lattice-preferred orientation in the mantle, contributing to seismic anisotropy (Jung and Karato, 2001; Jung et al., 2006). It should thus be possible to observe changes in the fabric of mantle rocks in places that have experienced fluid addition. Knowledge of seismic wave velocities in the mantle wedge is quite good, but seismic measurements of attenuation lag behind in resolution and accuracy, thus limiting our understanding of the mantle wedge rheology. Study of HP and UHP rocks will provide valuable insights into how fluids modify the mantle wedge by enabling estimates of the volume of fluid added, and the length scales and patterns of this addition. These insights will aid geophysicists, geochemists, and experimentalists by narrowing the parameter space currently confounded by a poorly known quantity and distribution of fluid.
- What is the mass fraction contributed by the mantle wedge during the generation of arc basalts (4.4, 4.5)?
Models for arc basalt petrogenesis attempt to reconstruct magma compositions by summing together a mantle-derived component and one or more slab-derived components (McCulloch and Gamble, 1991; Stolper and Newman, 1994; Ayers, 1998; Grove et al., 2002; Kimura et al., 2009), according to some mass fraction usually estimated by fitting the calculation to natural arc basalt data. The uncertainty in these calculations is very high, as neither the end-member compositions nor the mass fractions can be directly assessed. Direct observation of mantle that has been depleted by arc basalt extraction, and perhaps metasomatized by a slab-derived component, may provide some constraint on the endmembers contributing to this process. Direct samples of the mantle wedge are rare but they exist in the form of xenoliths in volcanic rocks from the Sierra Nevada (Ducea and Saleeby, 1998; Chin et al., 2012), the Mexican Volcanic Belt (Blatter and Carmichael, 2006), and South America (Rodriguez-Vargas et al., 2005).
- How does the mantle wedge evolve temporally in response to fluid addition and melt extraction (4.2, 4.6)?
In the early stages of arc initiation, the volcanic products of the arc include MORB-like forearc basalts and extremely depleted, high-Mg magmas commonly referred to as boninites, the latter representing high melt fractions (Hickey and Frey, 1982; Stern and Bloomer, 1992; Reagan et al., 2010). As the mature arc is established, these magmas evolve into common arc basalts, which are less depleted overall, and preferentially enriched in some elements – melt fractions have decreased, and mantle alteration by slab input becomes more evident. Ophiolites obducted onto the continents represent stages in the life cycle of a subduction zone from initiation to maturation (Shervais, 2001), providing direct, observable evidence for the evolution of the supra-subduction zone mantle through time. Moreover, progressive melt extraction and channelization in the mantle wedge provides an opportunity to see how melts evolve with time as the magmatic system matures in a developing arc mantle wedge. Large mantle massifs like the Josephine Peridotite and the Trinity Ophiolite in the western US offer the opportunity to observe the residues of melting, melt migration pathways, and how the associated melts have evolved through time both through physical observations of outcrop and regional scale features, and by chemical analysis of major and trace elements in residues and trapped melt products preserved in mantle veins and segregations in dunite pods and mantle shear zones (Kelemen et al., 1992; Kelemen and Dick, 1995).
- What are melt extraction rates and fluid fluxes through the wedge? Are fluxes uniform, in pulses, channelized, in diapirs (4.4)?
Evidence from U-series nuclides in arc basalts indicates that melt extraction from the mantle is extremely rapid, requiring extraction via channelized flow (Turner et al., 2001; Yokoyama et al., 2003). Direct observation of mantle rocks provides field evidence supporting the existence of melt extraction channels (Dick, 1977; Quick, 1981; Dick and Natland, 1993). Dunite formations observed in ophiolites from Oman are believed to represent similar channels formed beneath a mid-ocean ridge (Kelemen et al., 2000) and, significantly, provides scaling constraints for numerical models of melt extraction features (Spiegelman and Kelemen, 2003). Taken together with advances in seismic imaging, instrumentation, and the increasing availability of large datasets, the addition of field-based constraints on melt channelization should take us much closer to an understanding of melt transport mechanisms in the mantle.
- How prevalent is serpentinization, and what are the properties of serpentinite (i.e., antigorite) at the slab/mantle interface (4.4)?
Serpentine minerals (along with sheet silicates such as chlorite and talc) may help “lubricate” the subduction interface, and are likely a major mineralogical host for slab-derived fluids released at shallow depths, as well as the source of a small, but important menu of “fluid-mobile” elemental and isotopic tracers mobilized early from slabs (Leeman 1996; Benton et al 2001; Straub and Layne 2002; Morris and Ryan 2003). Serpentine breakdown is thus an important, if poorly understood, step in the transfer of fluid-hosted species from the downgoing plate into upper plate assemblages and arc magmas, and questions as to whether wedge-derived or slab-derived serpentinites are the likely source of fluid-mobile species are being actively debated. Ocean Drilling Project (ODP) Legs 125 and 195 directly sample shallow, chrysotile/lizardite dominated serpentinites forming in the shallow IBM forearc mantle wedge. However, we have not been able to directly sample serpentinites formed in most other subduction zones, and in practice we have no access to serpentine assemblages formed under the P-T conditions of the slab-mantle interface, or within deep downgoing plates (conditions under which antigorite is likely the dominant serpentine variant). Examinations of exhumed, antigorite-dominated subduction zone serpentinites from the Franciscan, Syros, and in other preserved subduction mélange sequences, as well as olivine-dominated ultramafic assemblages that may have developed via serpentinite decomposition (e.g., chlorite-bearing peridotites of the Pyrenees) can place constraints on the chemical pathways of slab-derived species through the subduction interface and mantle wedge (e.g., Penniston-Dorland et al., 2010; Miller, et al., 2009). Studies in preserved forearc sites reflecting different P-T trajectories can help place constraints on the prevalence of serpentinization in subduction settings (i.e., is the forearc wedge always serpentinized, or are there variations associated with thermal structure?).
- What is the mass balance of carbon through the subduction system? How much carbon is sequestered in the mantle wedge via carbonation and serpentinization, and what is the ultimate fate of this subducted carbon (4.4)?
There has been considerable recent interest in characterizing the cycling of carbon at subduction zones through study of seafloor lithologies subducting into modern margins (sediment, altered oceanic basalts, and carbonated ultramafic rocks), subduction-zone metamorphic suites representing ancient carbon subduction, and exposed ophiolite sequences. This work can dovetail with study of carbon (and nitrogen) emissions in arc volcanic gases (see Halldorsson et al., 2013), in some cases at margins for which off-trench drilling allows estimation of the carbon inventory initially subducting at that margin (see Li and Bebout, 2005; de Leeuw et al., 2007). Peridotite alteration on the seafloor around slow spreading mid-ocean ridges leads to a carbon flux of 1 Mt C/yr (Kelemen et al., 2011; Alt et al., 2013), about 10% of that estimated for alteration of shallow volcanic rocks in the same setting (Alt and Teagle, 1999). Based on seismic surveys indicating serpentinization of the oceanic mantle just before subduction, due to plate flexure, an additional flux of up to 1 Mt C/yr is estimated (Alt et al., 2013), while other estimates (Falk and Kelemen, 2013; Kelemen, 2013) are about ten times as large.
There is evidence for C retention in the subducting slab, based on evidence from subduction-related metasedimentary and metabasaltic rocks (Bebout et al., 2013b), and it appears that significant decarbonation in such oceanic crust sections may be driven by infiltration of the rocks by H2O-rich fluids evolved elsewhere in the subducting slab (e.g., from nearby dehydrating rocks or from sub-crustal hydrated ultramafic rocks; see also Gorman et al., 2006). Based on studies of prograde metamorphism of oceanic mantle serpentinites, it is inferred that there is no loss of carbon from the subducting oceanic mantle section during subduction to sub-arc depths (Alt et al., 2012). Fully carbonated peridotites in Oman, formed along the subduction zone carrying the mantle section of the Samail ophiolite over metasedimentary rocks at about 100°C, indicate that mass transfer in this setting could represent a global flux of carbon of about 0.05 Mt/yr from compacting sediments into the “leading edge of the mantle wedge” (Falk and Kelemen, 2013; Kelemen, 2013). Deeper in subduction systems, carbon solubility in aqueous fluids interacting with subducting sediment increases dramatically (Dolejs and Manning, 2010; Manning, 2013; Manning et al., 2013). Global carbon fluxes from aqueous fluid-fluxed sediments into the hanging wall could reach 10 Mt/yr, and this estimate is roughly consistent with inferred carbon uptake in the partially serpentinized, “cold nose” of the mantle wedge observed above subduction zones worldwide (Falk and Kelemen, 2013; Kelemen, 2013). Additional carbon sinks could include arc and continental lower crust (Manning and Ingebritsen, 2013). Incorporation of the potential carbon fluxes and reservoirs described here could have a substantial impact on estimated net fluxes in subduction zones, potentially balancing net inputs and outputs (see Dasgupta and Hirschmann, 2010; Bebout, 2014). However, a paucity of data on carbon concentration, as a minor element in oceanic mantle peridotites, mantle wedge peridotites, and both arc and continental lower crust, renders such hypotheses extremely speculative. This could be a fertile topic for future research.
One of the great strengths of GeoPRISMS and its predecessor MARGINS is the interdisciplinary nature of these programs. GeoPRISMS involves geoscientists from a wide range of disciplines including geology, geophysics, petrology, and geochemistry, and employs a diverse set of research methodologies including experiments, numerical modeling, and field studies. ExTerra seeks to support the collaborative, interdisciplinary aspect of GeoPRISMS by facilitating communication among this richly varied consortium of geoscientists. The proposed field institutes provide the opportunity for non-field geologists such as experimentalists, modelers, and seismologists to observe exhumed rocks in their natural state, thus creating an environment for exchange of ideas between field geologists and non-field geologists. Another proposal is to hold focused webinars on topics related to the study of exhumed terranes. These virtual workshops will encourage discussion among geoscientists approaching the study of subduction zones from a variety of disciplines and approaches.
Another way in which we can unify the diverse range of geoscientists involved in GeoPRISMS and ExTerra is through centralized sample and data management. One of the challenges in studying exhumed terranes is the general lack of any one location at which the entire subduction zone can be observed. By providing access to a core set of samples and data to all participants, we can better ensure a self-consistent picture of the subsurface of subduction zones while encouraging cross-pollination of ideas across disciplines.
Focused, interdisciplinary field institutes will bring together researchers from diverse backgrounds and initiate an interdisciplinary thought process with respect to the subsurface at subduction zones. The field institute is modeled after the Ocean Drilling Project approach, wherein scientists will travel together to map, discuss, and collect samples that will contribute to the community effort towards understanding active subduction zone processes. Field institutes will explore specific science questions identified by or consistent with the ExTerra White Paper (this document) and the GeoPRISMS SCD Science Plan. Presentations and discussion before and after field institutes will familiarize all participants with the geologic setting, introduce the scientific questions to be addressed, and explore the range of potential approaches to post-collection analysis. Student participation in field institutes may be facilitated by on-site pre-fieldtrip symposia, during which students will receive additional background information regarding subduction zone anatomy and dynamics, exhumed terranes, and the motivation behind the specific scientific questions to be explored during the field institute. Field workshops could also include a culminating 1-2 day meeting in a small local conference venue providing participants valuable time to summarize group observations, identify key tasks, and establish clear goals to carry meaningful research onwards towards a productive (i.e. published) outcome. Field institutes would typically run every other year, with participants meeting in interim years to work together on synthesis of data and manuscript writing. Field institutes and workshops will be leveraged with existing programs and/or affiliated with conferences, such as the GSA Field Forum, Penrose Conference, Goldschmidt Conference, or International Geoscience Programme (IGCP). Joining forces with international organizations such as the Zooming In Between Plates (ZIP) Project will foster international exchange of ideas and maximize the impact of workshops and fieldtrips. Below are a few examples of proposed field trips compatible with these goals.
- Santa Lucia Mountains, California
Convenors: Mihai Ducea, Alan Chapman, Jeremy Hourigan
- How is magma generated, stored, and transported in the crust?
- What is the mode of emplacement of metasedimentary rocks in arc lower crust (~1 GPa)? Are they gradually buried, thrust laterally into the crust, or diapirically emplaced from below?
- How variable is the bulk composition, fabric, melt/fluid content, and thermal structure of arc crust, and how might these properties influence our interpretation of seismic velocity profiles?
- What is the extent of exchange between arc magmas and arc crust? How do cumulates, restites, and wallrocks influence arc magma evolution?
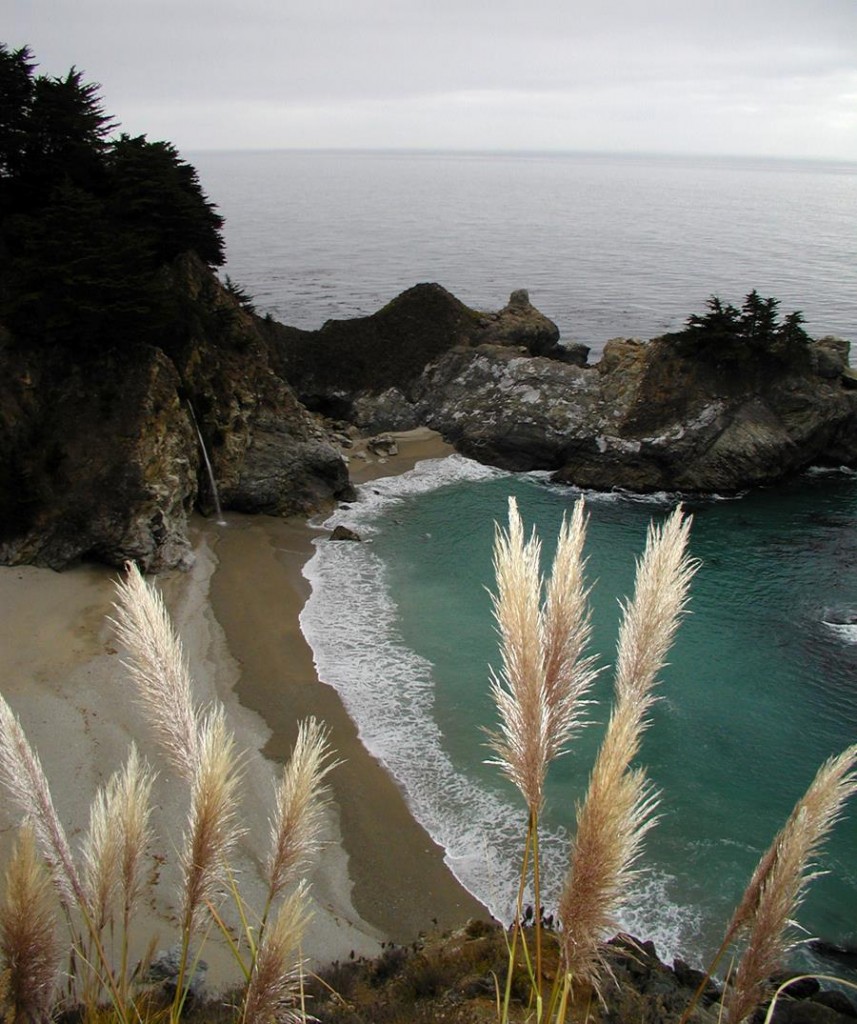
Figure 1. Deep crustal exposure of the Salinian Coast Ridge Belt at McWay’s fault, Santa Lucia Mountains. Photo credit: Mihai Ducea
Three blocks representing different parts of the subduction system are structurally juxtaposed within the Santa Lucia Mountains of California: the Nacimiento, Salinian, and Sierra de Salinas blocks. The Nacimiento block comprises classic Franciscan accretionary wedge rocks assembled in a tectonic melange, including some high-pressure/low-temperature rocks. The Salinian block is comprised of mid- to deep-crustal rocks of the late Cretaceous California magmatic arc, representing some of the deepest arc exposures in North America. The Sierra de Salinas block is a structural window into the accretionary wedge as it was shallowly subducted beneath the Salinian block during the Laramide orogeny. The field trip will focus particularly on the mechanisms of magmatic arc buildup and the structural aspects of tectonic underplating of accretionary wedge materials at the bottom of arc crust. A 5-6 day field institute in the Santa Lucia Mountains is proposed to examine these three critical components of the late Cretaceous Farallon-North America subduction system with 3 days of field observation, measurements, and sample collection, bracketed by an additional 2-3 days for presentations and discussion. This field trip would be run immediately before or after the 2014 Goldschmidt Conference in Sacramento, California, in order to minimize travel costs to participants.
- Catalina Schist, Santa Catalina Island, California
Convenors: Gray Bebout, Sarah Penniston-Dorland
- What is the nature and composition of the rocks at the interface between the downgoing subducting slab and the overlying mantle wedge and how do they change with depth?
- What are the pathways, fluxes and timescales of fluid release in the slab?
- What is the composition of slab-derived fluids and melts and how do these compositions change with depth?
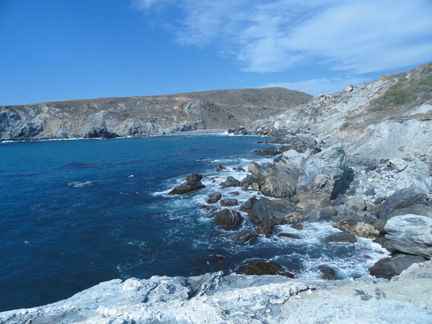
Figure 2. Tectonic blocks exposed along the coast of Santa Catalina Island, CA. Photo credit: Sarah Penniston-Dorland.
The Catalina Schist consists of exposures of sedimentary and oceanic crustal lithologies metamorphosed in an Early Cretaceous subduction zone. Units of widely varying peak metamorphic grade, but with similar protoliths, allow examination of devolatilization and other fluid-rock interactions as a function of varying prograde P-T history. Each of the units has an overall mélange fabric in which tectonic blocks and large tabular bodies of metasedimentary, metamafic, and metaultramafic rocks are embedded in a highly deformed and metasomatized matrix with hybridized compositions indicating mixtures of these rock types. The lithologic-structural character of the Catalina Schist is believed to reflect that in the subduction channel in modern subduction zones at depths of 15-45 km depths. The highest-grade unit of the Catalina Schist (amphibolite grade) shows evidence for partial melting of both mafic and sedimentary rocks, thus providing a glimpse into melt generation and mobility in hot subduction environments, whereas the lowest-grade units (lawsonite-albite and lawsonite-blueschist grade) contain rocks that experienced prograde P-T histories in colder subduction environments. Each unit contains abundant evidence for fluid-rock interactions and the higher-grade units have experienced extensive devolatilization resulting in release of particularly fluid-mobile trace elements. This field excursion would be aimed at demonstrating the overall structural, chemical, and lithological evolution of the subduction channel, and the nature of fluid-rock interactions as functions of metamorphic history/grade and structural position in these mélange units. In three days, it is possible to examine each of the tectonometamorphic units, in some detail, with ample time in the evenings for relevant presentations and discussions. The over-arching goal would be to consider the spatial-temporal evolution of the Catalina Schist and its relevance for understanding the range of structural, petrologic, and geochemical processes operating in modern forearcs, potentially affecting forearc seismicity and the inventory of materials reaching depths beneath arc volcanoes and to greater depths in the mantle.
- Fossil Subduction Interface, Western Alps
Convenor: Philippe Agard
- What is the nature and composition of the rocks at the interface between the downgoing subducting slab and the overlying mantle wedge and how do they change with depth?
- How is the slab signature transferred to the mantle? What is the relative importance of mechanical vs. chemical mixing?
- What can the rock record reveal about mechanical behavior associated with subduction-related seismic events?
- What are the pathways, fluxes and timescales of fluid release in the slab?
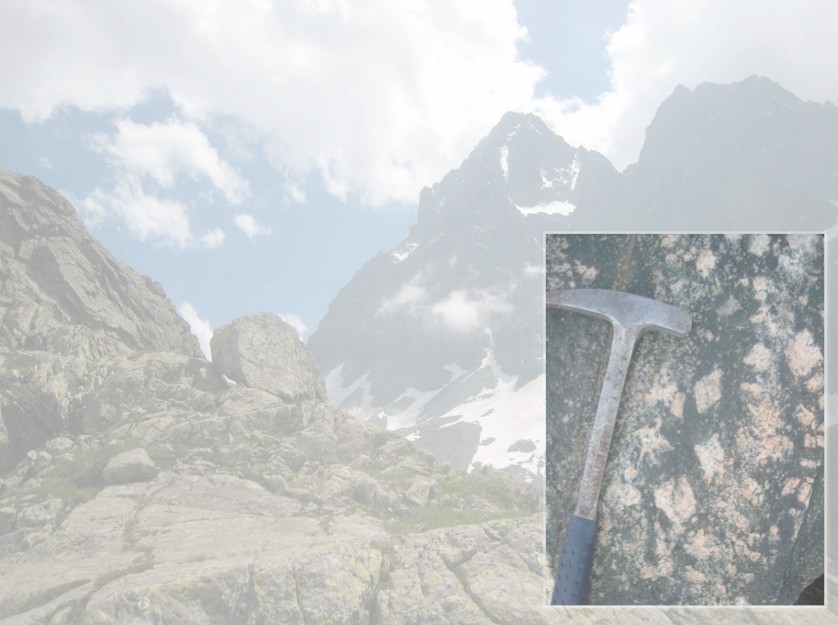
Figure 3. View of Monviso. Inset: large pseudomorphs afterlawsonite in blueschist. Photo credit: Philippe Agard.
This institute will focus on field observation of fossil plate interface from depths of 10 to nearly 100 km – at a range of scales from the mineral (and analytical) scale to the scale of the whole plate interface. The fieldtrip will provide the participants with the unique opportunity to study and walk down the fossil subduction interface, investigating remnants preserved from 10-15 km (Apennines) to 30-50 km (Dent Blanche) and finally 80 km depths (Monviso, Voltri), thereby realizing the potential nature and complexity of the subduction interface and the key proxies to consider when characterizing this geological environment. Special attention will be paid to observations of both petrology and structures (with focus on fluid and rheology) and scale transfer from the field to the subduction interface. In addition to the field traverse (~7 days), 3 days of indoor lecture will be organized.
- Accretionary Prism to Arc Root, South Island, New Zealand
Convenors: Harold Stowell, Joshua Schwartz, Keith Klepeis, Andy Tulloch, Nick Mortimer
- How variable is the bulk composition, fabric, melt/fluid content, and thermal structure of arc crust, and how might these properties influence our interpretation of seismic velocity profiles?
- How is magma generated, stored, and transported in the crust?
- What is the composition of slab-derived fluids and melts and how do these compositions change with depth?
- What are the pathways, fluxes and timescales of fluid release in the slab?
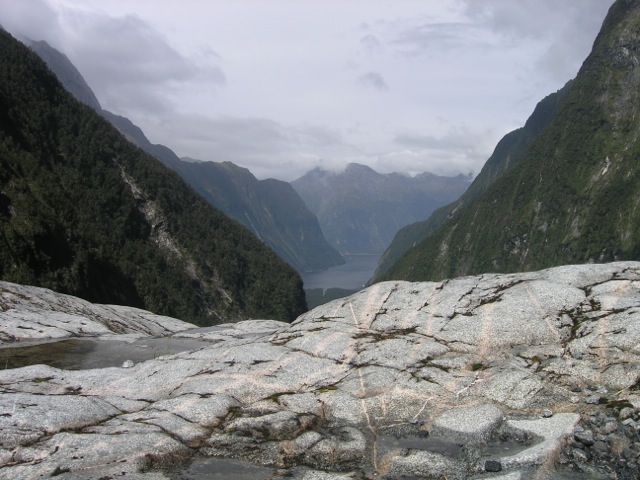
Figure 4. View toward Milford Village from Pembroke Valley, Fiordland. The Pembroke Granulite in foreground displays a rectilinear array of white to gray leucosomes and pink garnet reaction zones indicative of partial melting and wall rock reactions in the lower crust of the Cretaceous Gondwana Magmatic Arc. Photo credit: Harold Stowell.
The South Island of New Zealand includes excellent outcrops of a Mesozoic continental magmatic arc and its associated metamorphic rocks. Lower crustal sections of the arc are exposed in Fiordland and upper crustal sections of the same arc in Stewart Island. Collectively, these areas reveal that the middle to upper crustal levels of the Mesozoic Arc comprise large bodies of granodiorite and tonalite interlayered with metasedimentary rocks of greenschist metamorphic facies, and the lower crust is dominated by mafic igneous rocks interlayered with a sequence of pelitic gneisses and schists. To the east of the arc, low grade metamorphic rocks of the forearc basin and accretionary prism are exposed, which together with the Fiordland and Stewart Island sections, provide a near complete traverse across the arc at ca. 100 Ma. In Fiordland, a ca. 5000 km2 exhumed section of lower crust is exposed on the southeast side of the Alpine Fault. Exposed rocks include older (>130 Ma) low Sr/Y and younger (125-110 Ma) high Sr/Y plutonic belts which intruded amphibolite-facies metamorphic rocks at 20-45 km depth during subduction beneath the Gondwana margin. Plutons and host rocks were metamorphosed to granulite facies during the Early Cretaceous immediately post-dating a surge of mafic to intermediate magmatism. Subsequent extension and exhumation exposed them in several broad domes that are enveloped by amphibolite-facies ductile shear zones. These shear zones juxtapose the eclogite- and granulite-facies orthogneisses with host rocks and contain evidence for the extensional collapse of the orogen prior to continental breakup at c. 85 Ma. In Stewart Island and Nelson, plutons contemporaneous with those in Fiordland intrude the mid-upper crust. The igneous rocks include plutons that have been interpreted as upper crustal parts of the magmatic system exposed in Fiordland. Mid-upper level detachment faults in the Paparoa and Sisters metamorphic core complexes preserve a record of upper crustal extensional processes that occurred in concert with ductile deformation at deep crustal conditions in Fiordland. In Otago and Southland, the forearc basin and accretionary prism to the Mesozoic Arc are preserved. The forearc basin is a structurally simple zeolite facies synclinorium. The accretionary wedge comprises a c. 150 km-wide prehnite-pumpellyite to greenschist facies schist anticlinorium that shows progressive development of deformation fabrics and evidence for slab-derived fluid flux. Evidence for fluid flow includes numerous veins which are locally associated with gold deposits marking the locus of fluid flow of the large scale orogenic hydrothermal systems. The toe of the wedge extends north for 200 km as imbricated greywackes. The field trip would require ~12 days.
- Sierra Valle Fértil, Argentina
Convenors: Juan Otamendi and George Bergantz
- How is magma generated, stored, and transported in the crust?
- How variable is the bulk composition, fabric, melt/fluid content, and thermal structure of arc crust, and how might these properties influence our interpretation of seismic velocity profiles?
- What is the extent of exchange between arc magmas and arc crust? How do cumulates, restites, and wallrocks influence arc magma evolution?
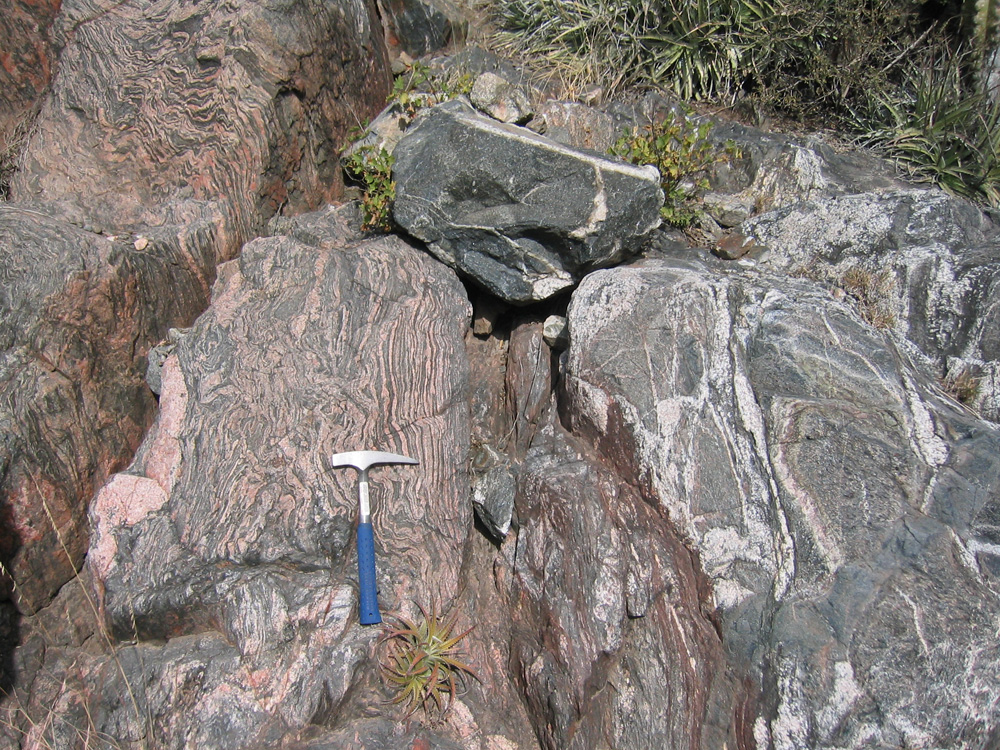
Figure 5. Contact between upper mafic complex and metasediments forming stomatic migmatites with leucogranite, and back-veining into gabbro from the Sierra Valle Fértil. Photo credit: George Bergantz.
The Sierra Valle Fértil – La Huerta section of the Famatina arc in west central Argentina exposes the plutonic roots and associated host rocks in a 130 km long, nearly continuous section with paleo-depths of >25 km to 8 km or less. This tilted section exposes identifiable end-member components of the continental arc: large bodies of granodiorite and tonalites in the mid-to-upper crust, a mafic- and ultramafic- dominated lower crust, which is interlayered with a lithologically homogenous sequence of metapelites and other supracrustal compositions. The “circulatory system” of the arc is continuous and decipherable: all units described above are physically linked via hyper-solidus and migmatitic domains, veins and dikes that connect source rocks to plutons. The preservation is exceptional, as arc magmatism was largely arrested by a soft collision during the prograde peak, before long-lasting magmatism had obliterated the architecture of a complete “compositional cycle” forming a voluminous mafic complex and a granodiorite batholith, in a span of 4-5 My. Hence much as volcanic systems are a ‘snapshot’ of a magmatic system, the Sierra Valle Fértil provides a ‘snapshot’ of a juvenile but crustal-scale plutonic system, without significant overprint of high temperature structures and very long-lived repeated magmatic processing. The field trip would examine a diverse variety of high-temperature assimilation styles, the homogenization and subsequent transport of fractionated products and their associated cumulates, as well as km-thick sections of mafic to ultra-mafic cumulates. The abrupt transitions between gabbro, tonalite, and granodiorite can been seen directly in the field, and the changing rheological conditions in both the highly melted host rock and mushy magmatic domains control homogenization over a variety of length scales. The field trip would require 7-8 days, consisting of 4 – 5 days in the field, two days of presentations and discussion, and a day of transport from Mendoza and back.
- Josephine Peridotite, Oregon / California
Convenors: John Shervais, Henry Dick
- How does the mantle wedge evolve temporally in response to fluid addition and melt extraction?
- What are melt extraction rates and fluid fluxes through the wedge? Are fluxes uniform, in pulses, channelized, in diapirs?
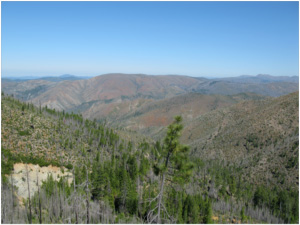
Figure 6. The Josephine Peridotite. Photo credit: Henry Dick.
The Josephine Peridotite is a ~700 km2 peridotite massif in southwestern Oregon and Northern California in the hanging wall of the coast range thrust. Presently overlying shales and greywackes of the Dothan Formation, and overlain structurally by the Rogue Volcanics and Galice shales and greywackes it is evident that this massif represents an enormous exposure of a mantle wedge that overlay an active subuction zone in the late Jurassic. The massif is easily reached by logging roads extending from Brookings, Oregon to its western margin. There, in the vicinity of Vulcan Peak on the edge of the Kalmiopsis Wilderness features related to late-stage melt migration through the mantle wedge in an arc-related magmatic event are beautifully exhibited. These include dunite bodies where melt flow was focused through the mantle to the overlying crust. Many of these bodies are found along high temperature fault zones where local melt segregations can be seen that crystallized to form gabbro and gabbronorite. The field trip would examine a wide range of features related to concurrent faulting and melt flow in the mantle wedge, and the process of melt-rock reaction that may significantly influence the geochemistry of early arc-related magmas. The excursion would take two days and two nights in the field, with another two days spent for discussions and presentations related fluid flow and mantle melting in arc-related environments at an appropriate setting in Northern California or Southwestern Oregon.
An interactive database will allow field geologists who collect samples to connect with those who have use for rock samples, which may include experimentalists, analytical geochemists, or any researchers who cannot participate in fieldwork due to health, time, or financial limitations. Samples collected during the ExTerra Field Institutes will be registered with International GeoSample Numbers (IGSN) via the System for Earth Sample Registration (SESAR; http://www.geosamples.org/), and will form the foundation of an integrated sample set and database, allowing researchers who could not attend the field seminar to reap the benefits of these ventures via access to both data and samples for original research. We propose an IODP-inspired model for sample sharing, whereby Field Institute participants would be given priority access for some period of time (~18 months), after which the samples would be made available to the community at large. Personal and institutional sample collections could also be shared using the proposed data and sample management system, enabling us to obtain regional coverage over larger areas. Many of the key players in the 1980s “golden age’ of metamorphic petrology in the United States are now retired or approaching retirement, and the ExTerra sample and data management system provides a way for these scientists to put the products of their lives’ work to good use, rather than allow them to collect dust in a storage space or on a hard drive. Several members of the ExTerra community have expressed interest in making their personal collections available by means of such a program.
The existing interactive database MetPetDB (http://www.metpetdb.rpi.edu) would serve as the foundation for the ExTerra integrated database and sample repository, and we will make use of the existing cyberinfrastructure, as MetPetDB already has the ability to handle most of the data types we would produce (see Figure 5). Users can choose whether they want their data to be viewable by anyone, or only by specified collaborators, allowing contributors to maintain control over their own data.
The establishment of a physical sample repository is somewhat more complicated, as it would require ongoing curation beyond the scope of GeoPRISMS. This is a timely and recurrent issue that has been brought up in the recent EarthCube Domain End-user workshops such as the Petrology and Geochemistry workshop held at the Smithsonian Institution in March, 2013. It is widely believed, and critical to the purposes of ExTerra, that samples collected in the field represent the “Level Zero” data of our science. ExTerra will pursue options for long-term sample management, using samples collected during Field Institutes as a starting point for building a repository or collection. There are at least two distinct models for sample management: either a curated collection (i.e., the Smithsonian model), whereby samples are assessed on the basis of their intrinsic scientific and historic value and chosen selectively for inclusion, versus a core repository model (i.e., the IODP model), where splits of all samples are kept and managed under the assumption that different characteristics might be sought by different end-users at different points in time. Both models have benefits and drawbacks, and we will work to develop the best model to suit the needs of the community with special consideration for sustainability.
Figure 7. Schematic showing data types (in blue type) associated with various levels of the sampling and field campaigns. Samples and sub-samples will be registered with parent and daughter IGSNs. MetPetDB currently possesses, will possess, or can be linked to other databases with the capacity to store all of these data types.
Virtual workshops and webinars are another way to bring together researchers from across disciplines, with the added benefit of cutting back on the considerable carbon footprint of our community. Topics will be solicited from the community, and may include such topics as:
- Relating petrology and seismic wave velocities
- The importance of starting materials for experimental petrology and rock mechanics
- Development and implementation of MetPetDB
- What is the 80 km coupling boundary and why is it important?
- Erosion, delamination, and evolution of the crust
Abers, G. A. (2005). Seismic low-velocity layer at the top of subducting slabs; observations, predictions, and systematics. Physics of the Earth and Planetary Interiors 149, 7-29.
Abers, G. A., van Keken, P.,E., Kneller, E. A., Ferris, A., Stachnik, J. C. (2006). The thermal structure of subduction zones constrained by seismic imaging; implications for slab dehydration and wedge flow. Earth and Planetary Science Letters 241, 387-397.
Agard, P., Yamato, P., Jolivet, L., Burov, E. (2009). Exhumation of oceanic blueschists and eclogites in subduction zones: timing and mechanisms. Earth-Science Reviews 92, 53-79.
Allan, M. M., Yardley, B. W. D., Forbes, L. J., Shmulovich, K. I., Banks, D. A., Shepherd, T. J. (2005). Validation of LA-ICP-MS fluid inclusion analysis with synthetic fluid inclusions. American Mineralogist 9, 1767-1775.
Alt, J. C., Garrido C. J., Shanks, W. C., Turchyn. A., Padrón-Navarta. J. A., et al. (2012). Recycling of water, carbon, and sulfur during subduction of serpentinites: A stable isotope study of Cerro del Almirez, Spain. Earth and Planetary Science Letters 327-328, 50–60.
Alt, J. C, Schwarzenbach, E. M., Früh-Green, G. L., Shanks, W. C., Bernasconi, S. M., et al. (2013). The role of serpentinites in cycling of carbon and sulfur: Seafloor serpentinization and subduction metamorphism. Lithos 178, 40-54.
Alt, J. C., Teagle, D. A. H. (1999). The uptake of carbon during alteration of oceanic crust. Geochimica et Cosmochimica Acta 63, 1527-1535.
Annen, C., Blundy, J., Sparks, R. S. (2006). The genesis of intermediate and silicic magmas in deep crustal hot zones, Journal of Petrology 47, 505-539.
Angiboust, S., Agard, P., Jolivet, L., Beyssac, O. (2009) The Zermatt-Saas ophiolite: the largest (60 km-wide) and deepest (c.70-80 km) continuous slice of oceanic lithosphere detached from a subduction zone? Terra Nova 21, 171-180.
Angiboust S., Agard, P., Yamato, P., Raimbourg, H. (2012) Eclogite breccias in a subducted ophiolite: a record of intermediate-depth earthquakes? Geology 40, 707-710.
Arndt, N. T., Goldstein, S. L. (1989). An open boundary between lower continental crust and mantle: its role in crust formation and crustal recycling. Tectonophysics 161, 201-12.
Atherton, M. P., Petford, N. (1993). Generation of sodium-rich magmas from newly underplated basaltic crust. Nature 362, 144-146.
Ayers, J. (1998). Trace element modeling of aqueous fluid; peridotite interaction in the mantle wedge of subduction zones. Contributions to Mineralogy and Petrology 132, 390-404.
Bachl, C. A., Miller, C. F., Miller, J. S., Faulds, J. E. (2001). Construction of a pluton: Evidence from an exposed cross section of the Searchlight pluton, Eldorado Mountains, Nevada. Geological Society of America Bulletin 113, 1213-1228.
Barruol, G., Kern, H. (1996) Seismic anisotropy and shear-wave splitting in lower-crustal and upper-mantle rocks from the Ivrea Zone; experimental and calculated data. Physics of the Earth and Planetary Interiors 95, 175-194,
Barth, A. P., Wooden, J. L., Tosdal, R. M., Morrison, J., Dawson, D. L., Hernly, B. M. (1995) Origin of gneisses in the aureole of the San Gabriel anorthosite complex and implications for the Proterozoic crustal evolution of Southern California.
Baxter, E.F. (2003). Natural constraints on metamorphic reaction rates in Vance, D., Mueller, W., Villa, I.M., eds., Geochronology; linking the isotopic record with petrology and textures. Geological Society Special Publications 220, 183-202.
Baxter E. F., Caddick, M. J., (2013). Garnet growth as a proxy for progressive subduction zone dehydration. Geology 41, 643-646.
Beane R., Wiebe R. (2012). Origin of quartz clusters in Vinalhaven granite and porphyry, coastal Maine. Contributions to Mineralogy and Petrology 163, 1069-1082.
Bebout, G.E. (1991). Field-based evidence for devolatilization in subduction zones – implications for arc magmatism. Science 4992, 413-416.
Bebout, G.E. (1997). Nitrogen isotope tracers of high-temperature fluid-rock interactions: Case study of the Catalina Schist, California. Earth and Planetary Science Letters. 151, 77-90.
Bebout, G. E., Barton, M. D. (1989). Fluid flow and metasomatism in a subduction zone hydrothermal system; Catalina Schist Terrane, California. Geology 17, 976-980.
Bebout, G.E., Barton, M.D. (2002). Tectonic and metasomatic mixing in a high-T subduction zone mélange – insights into the geochemical evolution of the slab-mantle interface. Chemical Geology 187, 79-106.
Bebout, G. E. (2012). Chapter 9. Metasomatism in subduction zones of subducted oceanic slabs, mantle wedges, and the slab-mantle interface, invited chapter, in Harlov, D., and Austrheim, H., eds., Metasomatism and the Chemical Transformation of Rock, The Role of Fluids in Terrestrial and Extraterrestrial Processes, Springer-Verlag, p. 289-349.
Bebout, G. E. (2014). Chemical and isotopic cycling in subduction zones. in The Crust, Vol. 4, Treatise on Geochemistry, (H.D. Holland and K.K. Turekian, eds.), ed. R. L. Rudnick, pp. 703-747. Oxford: Elsevier-Pergamon.
Bebout, G. E., Agard, P., Kobayashi, K., Moriguti, T., Nakamura, E. (2013a). Devolatilization history and trace element mobility in deeply subducted sedimentary rocks: SIMS evidence from Western Alps HP/UHP suites, Chemical Geology 342, 1-20.
Bebout, G. E., Collins, N., Cook-Kollars, J., Angiboust, S., Agard, P., Scambelluri, M., John, T., Kump, L. R. (2013b). Subduction-zone metamorphic pathway for deep carbon cycling: Evidence from the Italian Alps and the Tianshan, Fall AGU meeting, San Francisco, abstract number V13C-2629.
Behn, M. D., Kelemen, P. B. (2006). Stability of arc lower crust: Insights from the Talkeetna arc section, south central Alaska, and the seismic structure of modern arcs. Journal of Geophysical Research-Solid Earth 111, doi: 10.1029/2006JB004327.
Behn, M. D., Kelemen, P. B., Hirth, G., Hacker, B. R., Massonne, H. (2011). Diapirs as the source of the sediment signature in arc lavas. Nature Geoscience 4, 641-646.
Benton, L. D., Ryan, J. G., Tera, F. (2001). Boron isotope systematics of slab fluids as inferred from a serpentine seamount, Mariana forearc. Earth and Planetary Science Letters 187, 273-282.
Blatter, D. L., Carmichael, I. S. E. (1998). Hornblende peridotite xenoliths from Central Mexico reveal the highly oxidized nature of subarc upper mantle. Geology 26, 1035-1038.
Bostock, M. G., Hyndman, R.D., Rondenay, S., Peacock, S.M. (2002). An inverted continental Moho and serpentinization of the forearc mantle. Nature 417, 536–538.
Breeding, C. M., Ague, J. J. (2002) Slab-derived fluids and quartz-vein formation in an accretionary prism, Otago Schist, New Zealand. Geology 30, 499-502.
Brenan, J. M., Shaw, H. F., Ryerson, F. J. (1995). Experimental evidence for the origin of lead enrichment in convergent-margin magmas. Nature 378, 54-56.
Brown, E. H., Walker, N.W. (1993). A magma-loading model for Barrovian metamorphism in the southeast Coast Plutonc Complex, British Columbia and Washington. Geological Society of America Bulletin 105, 479-500.
Calvert, A. J., Klemperer, S. L., Takahashi, N., Kerr, B. C. (2008). Three-dimensional crustal structure of the Mariana island arc from seismic tomography. Journal of Geophysical Research 113, B01406. doi:10.1029/2007JB004939.
Chapman, A. D., Saleeby, J. B., Wood, D. J., Piasecki, A., Kidder, S., Ducea, M. N., Farley, K. A. (2012). Late Cretaceous gravitational collapse of the southern Sierra Nevada batholith, California. Geosphere 8, 314-341.
Chin, E. J., Lee, C.-T. A., Luffi, P., Tice, M. (2012) Deep lithospheric thickening and refertilization beneath continental arcs; case study of the P, T and compositional evolution of peridotite xenoliths from the Sierra Nevada, California. Journal of Petrology 53, 477-511.
Class, C., Miller, D. M., Goldstein, S. L., Langmuir, C. H. (2000). Distinguishing melt and fluid subduction and components in Umnak Volcanics, Aleutian Arc. Geochemistry, Geophysics, Geosystems 1, doi:10.1029/1999GC000010.
Cloos, M. (1982). Flow melanges; numerical modeling and geologic constraints on their origin in the Franciscan Subduction Complex, California. Geological Society of America Bulletin 93, 330-344.
Coldwell, B., Adam, J., Rushmer, T., Macpherson, C. G. (2011). Evolution of the East Philippine Arc; experimental constraints on magmatic phase relations and adakitic melt formation. Contributions to Mineralogy and Petrology 162, 835-848.
Cruz-Uribe, A., Feineman, M., Zack, T., Barth, M. (2014). Metamorphic reaction rates at ~650-800˚C from diffusion of Nb in rutile. Geochimica et Cosmichimica Acta, accepted.
Currie, C. A., Beaumont, C., Huismans, R. S. (2007). The fate of subducted sediments: A case for backarc intrusion and underplating. Geology 35, 1111-1114.
Dasgupta, R., Hirschmann, M. M. (2010). The deep carbon cycle and melting in Earth’s interior. Earth and Planetary Science Letters 298, 1-13, doi:10.1016/j.epsl.2010.06.039.
Davidson, J., Turner, S., Handley, H., Macpherson, C., Dosseto, A. (2007). Amphibole sponge in arc crust? Geology 35, 787-790.
de Leeuw, G. A., Hilton, D. R., Fischer, T. P., Walker, J. A. (2007). The He\CO2 isotope and relative abundance characteristics of geothermal fluids in El Salvador and Honduras: new constraints on volatile mass balance of the Central American Volcanic Arc. Earth and Planetary Science Letters 258, 132–146.
DeBari, S.M, Greene, A. R. (2011). Vertical stratification of composition, density, and inferred magmatic processes in exposed arc crustal sections. In: Brown, D., Ryan, P., (eds), Arc-Continent Collision, Frontiers in Earth Sciences, Springer-Verlag, Berlin, pp. 121-144.
DeBari, S. M., Sleep, N. H. (1991). High-Mg, low-Al bulk composition of the Talkeetna island arc, Alaska: implications for primary magmas and the nature of arc crust. Geological Society of America Bulletin 103, 37-47.
DeCelles, P. G., Ducea, M. N., Kapp, P., Zandt, G. (2009). Cyclicity in Cordilleran orogenic systems. Nature Geoscience 2, 251-257.
Deering, C. D., Bachmann, O., Vogel, T. A. (2011) The Ammonia Tanks Tuff: erupting a melt-rich rhyolite cap and its remobilized crystal cumulate. Earth and Planetary Science Letters 310, 518-525.
Deering ,C. D., Bachmann, O. (2010) Trace element indicators of crystal accumulation in silicic igneous rocks. Earth and Planetary Science Letters 297, 324-331.
Deering, C. D., Bachmann, O., Dufek, J., Gravley, D. M. (2011) Rift-related transition from andesite to rhyolite volcanism in the Taupo Volcanic Zone (New Zealand) controlled by crystal-melt dynamics in mush zones with variable mineral assemblages. Journal of Petrology 52, 2243-2263.
DePaolo, D.J. (1981). Trace element and isotopic effects of combined wallrock assimilation and fractional crystallization, Earth Planetary Science Letters 53, 189-202.
Dessimoz, M., Müntener, O., Ulmer, P. (2012). A case for hornblende dominated fractionation of arc magmas: the Chelan Complex (Washington Cascades). Contributions to Mineralogy and Petrology 163,567–589.
Dick, H. J. B. (1977) Evidence of partial melting in the Josephine Peridotite. In: H. J. B. Dick (ed), Bulletin – Oregon Dept. of Geology and Mineral Industries vol. 96, pp. 59-62.
Dick, H. J. B., Natland, J. R., Leg 147 Scientific Party (1993). An offset drilled mantle section: evidence for focused melt flow beneath the East Pacific Rise from ODP Leg 147. Geological Society of America, Abstracts with Programs 25, p. 444.
Dolejs, D., Manning, C. E. (2010). Thermodynamic model for mineral solubility in aqueous fluids: Theory, calibration and application to model fluid-flow systems. Geofluids 10, 20-40.
Dragovic, B., Samanta, L. M., Baxter, E. F., Selverstone, J. (2012). Using garnet to constrain the duration and rate of water-releasing metamorphic reactions during subduction: An example from Sifnos, Greece. Chemical Geology 314-317, p. 9-22.
Ducea, M. N. (2001). The California arc: Thick granitic batholiths, eclogitic residues, lithospheric-scale thrusting, and magmatic flare-ups. GSA Today 11, 4-11.
Ducea, M. N. (2002). Constraints on the bulk composition and root foundering rates of continental arcs: A California arc perspective. Journal of Geophysical Research 107, doi: 2001JB001643.
Ducea, M. N., Kidder, S., Chesley, J. T., Saleeby, J. B. (2009). Tectonic underplating of trench sediments beneath magmatic arcs: the central California example. International Geology Review 51, 1-26.
Ducea, M. N., Kidder, S., Zandt, G., (2003). Arc composition at mid-crustal depths: Insights from the Coast Ridge Belt, Santa Lucia Mountains, California. Geophysical Research Letters 30, doi: 2002GL16297.
Ducea, M. N., Otamendi, J. E., Bergantz, G., Stair, K. M., Valencia, V. A., Gehrels, G. E. (2010). Timing constraints on building an intermediate plutonic arc crustal section: U- Pb zircon geochronology of the Sierra Valle Fertil-La Huerta, Famatinian Arc, Argentina. Tectonics 29, TC4002, doi:10.1029/2009TC002615.
Ducea, M. N., Saleeby, J.B. (1996). Buoyancy sources for a large unrooted mountain range, the Sierra Nevada, California: Evidence from xenolith thermobarometry: Journal of Geophysical Research 101, 8229-8241.
Ducea, M. N., Saleeby, J. B. (1998). The age and origin of a thick mafic ultramafic root from beneath the Sierra Nevada batholith: Contributions to Mineralogy and Petrology 133, 169-185.
Ducea, M. N., Saleeby, J. B. (1998). A case of delamination of the deep batholithic crust beneath the Sierra Nevada, California. International Geology Review 40, 78-93.
Ducea, M. N., Seclaman, A. C., Murray, K. E., Jianu, D., Schoenbohm, L. M. (2013) Mantle-drip magmatism beneath the Altiplano-Puna plateau, central Andes, Geology 41, 915-918, doi: 10.1130/G34509.1.
Dufek, J., Bergantz, G. (2005). Lower crustal magma genesis and preservation: a stochastic framework for the evaluation of basalt–crust interaction. Journal of Petrology 46, 2167-2195.
Dufek, J., Bachmann, O. (2010). Quantum magmatism: Magmatic compositional gaps generated by melt-crystal dynamics. Geology 38, 687-690.
Ellam, R. M., Hawkesworth, C. J. (1998) Elemental and isotopic variations in subduction related basalts: evidence for a three-component model. Contributions to Mineralogy and Petrology 98, 72-80.
Elliott, T., Plank, T., Zindler, A., White, W., Bourdon, B. (1997). Element transport from slab to volcanic front at the Mariana Arc. Journal of Geophysical Research, 102, 14,991-15,019.
Evans, B. W., Berti, J. W. (1986). A revised metamorphic history for the Chiwaukum schist, North Cascades, Washington. Geology 14, 695-698.
Falk, E. S., Kelemen, P. B. (2013). Fully carbonated peridotite (listvenite) from the Samail ophiolite, Oman. Fall Meeting AGU, San Francisco CA 9-13 Dec: MR22A-03.
Feineman, M. D., Ryerson, F. J., DePaolo, D. J., Plank, T. (2007) Zoisite-aqueous fluid trace element partitioning with implications for subduction zone fluid composition. Chemical Geology 239, 250-265.
Frezzotti, M. L., Selverstone, J., Sharp, Z.D., and Compagnoni, R. (2011). Carbonate dissolution during subduction revealed by diamond-bearing rocks from the Alps. Nature Geoscience 4, 10, 703-706.
Gao, J., John, T., Klemd, R., Xianming, X. (2007). Mobilization of Ti-Nb-Ta during subduction; evidence from rutile-bearing dehydration segregations and veins hosted in eclogite, Tianshan, NW China. Geochimica et Cosmochimica Acta, 71, 4974-4996.
Gehrels, G. E., Patchett, P. J., Pearson, D., Girardi, J., Ducea, M. N., et al. (2009). U-Pb geochronology of the Coast Mountains Batholith in north-coastal British Columbia: constraints on age and tectonic evolution. Geological Society of America Bulletin 121, 1341–1361.
Gerya, T. V., Yuen, D. A. (2003). Rayleigh-Taylor instabilities from hydration and melting propel “cold plumes” at subduction zones. Earth and Planetary Science Letters 212, 47-62.
Girardi, J. D., Patchett, P. J., Ducea, M. N., Gehrels, G. E., Cecil, M. R., Rusmore, M. E., Woodsworth, G. J., Pearson, D. M., Manthei, C., Wetmore, P. (2012). Elemental and Isotopic Evidence for Granitoid Genesis From Deep-Seated Sources in the Coast Mountains Batholith, British Columbia. Journal of Petrology 53, 1505-1536.
Gorman, P. J., Kerrick, D. M., Connolly, J. A. D. (2006). Modeling open system metamorphic decarbonation of subducting slabs: Geochemistry, Geophysics, Geosystems 7 (4), Q04007, doi:10.1029/2005GC001125.
Greene, A. R., DeBari, S. M., Kelemen, P. B., Blusztajn, J., Clift, P. D. (2006). A detailed geochemical study of island arc crust; the Talkeetna Arc section, South-Central Alaska. Journal of Petrology 47, 1051-1093.
Gromet, L. P., Silver, L. T. (1987) REE variations across the Peninsular Ranges Batholith; implications for batholith petrogenesis and crustal growth in magmatic arcs. Journal of Petrology 28, 75-125.
Grove, M., Bebout, G. E., Jacobson, C. E., Barth, A. P., Kimbrough, D. L., King, R. L., Zou, H., Lovera, O. M., Mahoney, B. J., Gehrels, G. E. (2008). The Catalina Schist: evidence for mid-Cretaceous subduction erosion of southwestern North America, in Formation and Applications of the Sedimentary Record in Arc Collision Zones (A. E. Draut, P. D. Clift, and D. W. Scholl, eds.), Geological Society of America Special Paper 436, 335-361.
Grove, T. L., Parman, S. W., Bowring, S. A., Price, R. C., Baker, M. B. (2002). The role of an H2O-rich fluid component in the generation of primitive basaltic andesites and andesites from the Mt. Shasta region, N California. Contributions to Mineralogy and Petrology 142, 375-396.
Gutierrez, F., Parada, M. A. (2010). Numerical modeling of time-dependent fluid dynamics and differentiation of a shallow basaltic magma chamber. Journal of Petrology 51, 731-762.
Hacker, B. R. (2008). H2O subduction beyond arcs, Geochemistry, Geophysics, Geosystems 9, Q03001, doi:10.1029/2007GC001707.
Hacker, B.R., Abers, G.A., Peacock, S.M. (2003) Subduction Factory 1. Theoretical mineralogy, densities, seismic wave speeds, and H2O contents. Journal of Geophysical Research 108, B1, 2029, doi:10.1029/2001JB00127.
Hacker, B. R., Kelemen, P. B., Behn, M. D. (2011) Differentiation of the continental crust by relamination. Earth and Planetary Science Letters 307, 501-516.
Hacker, B. R., Mehl, L., Kelemen, P. B., Rioux, M., Behn, M. D., Luffi, P. (2008). Reconstruction of the Talkeetna intraoceanic arc of Alaska through thermobarometry, Journal of Geophysical Research 113, B03204, doi:10.1029/2007JB005208.
Halldorsson, S. A., Hilton, D. R., Troll, V. R., Fischer, T. P. (2013). Resolving volatile sources along the western Sunda arc, Indonesia. Chemical Geology, http://dx.doi.org/10.1016/j.chemgeo.2012.09.042.
Herzberg, C. T., Fyfe, W. S., Carr, M. J. (1983). Density constraints on the formation of the continental Moho and crust. Contributions to Mineralogy and Petrology 84, 1-5.
Hickey, R. L., Frey, F. A. (1982). Geochemical characteristics of boninite series volcanics; implications for their source. Geochimica et Cosmochimica Acta 46, 2099-2116.
Hildreth, W., Moorbath, S. (1988). Crustal contributions to arc magmatism in the Andes of Central Chile. Contributions to Mineralogy and Petrology 98, 455-489.
Hirth, G., Kohlstedt, D. L. (1996). Water in the oceanic upper mantle; implications for rheology, melt extraction and the evolution of the lithosphere. Earth and Planetary Science Letters 144, 93-108.
Hirth, G., Kohlstedt, D. (2003). Rheology of the upper mantle and mantle wedge; a view from the experimentalists. In: Eiler, J. M. (ed), Inside the Subduction Factory, Geophysical Monograph 138, 83-105.
Humphreys, M. C. S. (2009). Chemical evolution of intercumulus liquid, as recorded in plagioclase overgrowth rims from the Skaergaard Intrusion. Journal of Petrology 50, 127-145.
Jackson, M. D., Gallagher, K., Petford, N., Cheadle, M. J. (2005). Towards a coupled physical and chemical model for tonalite-trondhjemite-granodiorite magma formation. Lithos 79, 43-60.
Jagoutz, O. E. (2010). Construction of the granitoid crust of an island arc. part II: A quantitative petrogenetic model. Contributions to Mineralogy and Petrology 160, 359-381.
Jagoutz, O., Behn, M. D. (2013) Foundering of lower island-arc crust as an explanation for the origin of the continental Moho. Nature 504, 131-134.
Jagoutz, O., Müntener, O., Schmidt, M. W., Burg, J. P. (2011). The roles of flux-and decompression melting and their respective fractionation lines for continental crust formation: Evidence from the Kohistan arc. Earth and Planetary Science Letters 303, 25-36.
Jagoutz, O., Schmidt, M. W. (2012). The formation and bulk composition of modern juvenile continental crust: The Kohistan arc. Chemical Geology 298, 79-96.
John, T., Gussone, N., Podladchikov, Y., Bebout, G., Dohmen, R., Halam, R., Klemd, R., Magna, T., Seitz, H-M. (2012). Volcanic arcs fed by pulsed fluid flow through subducting slabs. Nature Geoscience, doi: 10.1038/NGEO1482.
John, T., Schenk, V. (2006). Interrelations between intermediate-depth earthquakes and fluid flow within subducting oceanic plates: Constraints from eclogite facies pseudotachylytes. Geology 34, 557-560.
Jull, M., Kelemen, P. B. (2001). On the conditions for lower crustal convective instability. Journal of Geophysical Research 106, 6423-46.
Jung, H., Katayama, I., Jiang, Z., Hiraga, T., Karato, S. (2006). Effect of water and stress on the lattice-preferred orientation of olivine. Tectonophysics, 421(1-2), 1-22.
Jung, H., Karato, S. (2001). Water-induced fabric transitions in olivine. Science 293, 1460-1462.
Karato, S., Jung, H. (1998). Water, partial melting and the origin of the seismic low velocity and high attenuation zone in the upper mantle. Earth and Planetary Science Letters 157, 193-207.
Kay, R. W., Kay, S. M.. (1991). Creation and destruction of lower continental crust. Geologische Rundschau 80, 259-78.
Kay, S. M., Coira, B., Viramonte, J. (1994). Young mafic back arc volcanic rocks as indicators of continental lithospheric delamination beneath the Argentine Puna Plateau, central Andes. Journal of Geophysical Research 99(B12), 24323–24339, doi: 10.1029/94JB00896.
Kelemen, P. (2013). Some Less Conventional Processes in Subduction Zones. Mineralogical Magazine 77, 1444.
Kelemen, P. B., Braun, M., Hirth, G. (2000). Spatial distribution of melt conduits in the mantle beneath oceanic spreading ridges; observations from the Ingalls and Oman ophiolites. Geochemistry, Geophysics, Geosystems 1, doi:10.1029/1999GC000012.
Kelemen, P. B., Dick, H. J. B. (1995). Focused melt flow and localized deformation in the upper mantle: Juxtaposition of replacive dunite and ductile shear zones in the Josephine peridotite, SW Oregon. Journal of Geophysical Research 100, 423-438.
Kelemen, P. B., Dick, H. J. B., Quick, J. E. (1992). Formation of harzburgite by pervasive melt/rock reaction in the upper mantle. Nature 358, 635-641.
Kelemen, P. B., Hanghøj, K., Greene, A. (2004). One view of the geochemistry of subduction-related magmatic arcs, with an emphasis on primitive andesite and lower crust. In The Crust, Vol. 3, Treatise on Geochemistry, (H.D. Holland and K.K. Turekian, eds.), ed. R. L. Rudnick, pp. 593-659. Oxford: Elsevier-Pergamon.
Kelemen, P. B., Hanghøj, K., Greene, A. (2014). One view of the geochemistry of subduction-related magmatic arcs, with an emphasis on primitive andesite and lower crust. In The Crust, Vol. 4, Treatise on Geochemistry, (H.D. Holland and K.K. Turekian, eds.), ed. R. L. Rudnick, pp. 749-805. Oxford: Elsevier-Pergamon.
Kelemen, P. B., Matter, J., Streit, E. E., Rudge, J. F., Curry, W. B., Bluztajn, J. (2011). Rates and mechanisms of mineral carbonation in peridotite: Natural processes and recipes for enhanced, in situ CO2 capture and storage. Annual Reviews in Earth and Planetary Science 39, 545-76.
Kelemen, P. B., Rilling, J. L., Parmentier, E. M., Mehl, L., Hacker, B. R.. (2003). Thermal structure due to solid-state flow in the mantle wedge beneath arcs, Chapter 13. In Inside the Subduction Factory, AGU Monograph 138, ed. J. Eiler, pp. 293-311. Washington DC: American Geophysical Union.
Kelley, K. A., Cottrell, E. (2009). Water and the oxidation state of subduction zone magmas. Science 325, 605-607.
Kessel, R., Schmidt, M. W., Ulmer, P., Pettke, T. (2005). Trace element signature of subduction-zone fluids, melts and supercritical liquids at 120-180km depth. Nature, 437, 724-727.
Kidder, S., Ducea, M., Gehrels, G., Patchett, P. J., Vervoort, J. (2003). Tectonic and magmatic development of the Salinian Coast Ridge Belt, California. Tectonics 22, doi: 10.1029/2002TC001409.
Kim, Y., Clayton, R.W., Asimow, P.D., Jackson, J.M. (2013). Generation of talc in the mantle wedge and its role in subduction dynamics in central Mexico. Earth and Planetary Science Letters, 384, 81-87.
Kimura, J., Hacker, B. R., van Keken, P.,E., Kawabata, H., Yoshida, T., Stern, R. J. (2009). Arc Basalt Simulator version 2, a simulation for slab dehydration and fluid-fluxed mantle melting for arc basalts; Modeling scheme and application. Geochemistry, Geophysics, Geosystems 10, Q09004. doi:10.1029/2008GC002217.
King, R. L., Bebout, G. E., Kobayashi, K., Nakamura, E., van der Klauw, S.,N.G.C. (2004). Ultrahigh-pressure metabasaltic garnets as probes into deep subduction zone chemical cycling. Geochemistry, Geophysics, Geosystems 5, doi:10.1029/2004GC000746.
King, R. L., Bebout, G. E., Moriguti, T., Nakamura, E. (2006). Elemental mixing systematics and Sr-Nd isotope geochemistry of melange formation: Obstacles to identification of fluid sources to arc volcanics. Earth and Planetary Science Letters 246, 288-304.
King, R. L., Bebout, G. E., Grove, M., Moriguti, T., Nakamura, E. (2007). Boron and lead isotope signatures of subduction-zone melange formation: Hybridization and fractionation along the slab-mantle interface beneath volcanic arcs. Chemical Geology 239, 305-322.
Kitamura, K., Ishikawa, M., Arima, M. (2003). Petrological model of the Northern Izu-Bonin-Mariana arc crust; constraints from high-pressure measurements of elastic wave velocities of the Tanzawa plutonic rocks, Central Japan. Tectonophysics 371, 213-221.
Kodaira, S., Sato, T., Takahashi, N., Miura, S., Tamura, Y., Tatsumi, Y., Kaneda, Y. (2007). New seismological constraints on growth of continental crust in the Izu-Bonin intra-oceanic arc. Geology 35, 1031-1034.
Lee, C.-T., Rudnick, R. L., Brimhall, Jr., G. H. (2001) Deep lithospheric dynamics beneath the Sierra Nevada during the Mesozoic and Cenozoic as inferred from xenolith petrology. Geochemistry, Geophysics, Geosystems 2, 10.1029/2001GC000152, 2001.
Lee, C.-T. A., Morton, D. M., Little, M. G., Kistler, R., Horodyskyj, U. N., Leeman, W. P., Agranier, A. (2008). Regulating continent growth and composition by chemical weathering. Proceedings of the National Academy of Sciences of the United States of America 105, 4981-4986.
Leeman, W. P. (1996). Boron and other fluid-mobile elements in volcanic arc lavas; implications for subduction processes. In: Bebout, G.E. (ed), Subduction Top to Bottom, Geophysical Monograph 96, 269-276.
Levander, A., Schmandt, B., Miller, M. S., Liu, K., Karlstrom, K. E., Crow, R. S., Lee, C. T. A., Humphreys, E. D. (2011) Continuing Colorado Plateau uplift by delamination-style convective lithospheric downwelling. Nature 472, 461-465.
Li, L., Bebout, G. E. (2005). Carbon and nitrogen geochemistry of sediments in the Central American convergent margin: Insights regarding subduction input fluxes, diagenesis, and paleoproductivity. Journal of Geophysical Research, 110, B11202, doi:10.1029/2004JB003276.
Lloyd, G. E., Butler, R. W. H., Casey, M, Mainprice, D. (2009) Mica, deformation fabrics and the seismic properties of the continental crust. Earth and Planetary Science Letters 288, 320-328.
Lloyd, G. E., Butler, R. W. H., Casey, M., Tatham, D. J., Mainprice, D. (2011) Constraints on the seismic properties of the middle and lower continental crust. Geological Society of London Special Publications 360, 7-32.
Macpherson, C. G., Dreher, S. T., Thirlwall, M. F. (2006). Adakites without slab melting; high pressure differentiation of island arc magma, Mindanao, the Philippines. Earth and Planetary Science Letters 243, 581-593.
Manning, C. E. (1998). Fluid composition at the blueschist-eclogite transition in the model system Na2O-MgO-Al2O3-SiO2-H2O-HCl. Schweizer Mineralogische und Petrographische Mitteilungen 78, 225-242.
Manning, C. E. (2013). Thermodynamic modeling of fluid-rock Interaction at mid-crustal to upper-mantle conditions. Reviews in Mineralogy and Geochemistry 76, 135-64.
Manning, C. E., Ingebritsen, S. (2013). Diffuse degassing through magmatic arc crust. Fall Meeting AGU, San Francisco CA 9-13 Dec: V12B-02.
Manning, C. E., Shock, E. L., Sverjensky, D. A. (2013). The chemistry of carbon in aqueous fluids at crustal and upper-mantle conditions: Experimental and theoretical constraints. Reviews in Mineralogy and Geochemistry 75, 109-48.
Marschall, H. R., Schumacher, J. C. (2012) Arc magmas sourced from mélange diapirs in subduction zones. Nature Geoscience 5, 862-867.
Massonne, H-J. (2005). Involvement of crustal material in delamination of the lithosphere after continent-continent collision. International Geology Review 47, 792-804.
Massonne H-J. (2006). Early metamorphic evolution and exhumation of felsic high-pressure granulites from the north-western Bohemian Massif. Mineralogy and Petrology 86, 177-202.
Matzel, J. E. P., Bowring, S. A., Miller, R. B. (2008). Spatial and temporal variations in Nd isotopic signatures across the crystalline core of the North Cascades, Washington. Geological Society of America Special Papers 438, 499-561.
McCulloch, M. T., Gamble, A. J. (1991). Geochemical and geodynamical constraints on subduction zone magmatism. Earth and Planetary Science Letters 102, 358-374.
Miller, D. J., Christensen, N. I. (1994). Seismic signature and geochemistry of an island arc; a multidisciplinary study of the Kohistan accreted terrane, northern Pakistan. Journal of Geophysical Research, B, Solid Earth and Planets 99, 11,623-11,642.
Miller, D. P., Marschall, H. R., Schumacher, J. C. (2009). Metasomatic formation and petrology of blueschist facies hybrid rocks from Syros (Greece); implications for reactions at the slab-mantle interface. Lithos 107, 53-67.
Miller, R. B., Paterson, S. R., Matzel, J. P. (2009a). Plutonism at different crustal levels: Insights from the ~5–40 km (paleodepth) North Cascades crustal section, Washington, GSA Special Paper, v. 456, p. 125-149.
Miller, R. B., Gordon, S. M., Bowring, S. A., Doran, B. A., McLean, N. M., Michels, Z. D., Shea, E. K., Whitney, D. L., Wintzer, N. E., Mendoza, M. K., (2009b). Linking deep and shallow crustal processes in an exhumed continental arc, North Cascades, Washington, in O’Connor, J. E., Dorsey, R. J., Madin, I. P., eds., Volcanoes to Vineyards: Geologic Field Trips through the Dynamic Landscape of the Pacific Northwest: Geological Society of America Field Guide 15, p. 373–406, doi: 10.1130/2009.fl d015(19).
Morris, J. D., Ryan, J. G. (2003) Subduction zone processes and implications for changing composition of the upper and lower mantle. In: Carlson, R. (ed) Treatise on Geochemistry, Part 2: The Mantle, Chapter 2.11, pp. 451-470.
Mulcahy, S. R., King, R. L., Vervoort, J. D. (2009) Lawsonite Lu-Hf geochronology: A new geochronometer for subduction zone processes. Geology 37, 987-990.
Otamendi, J. E., Ducea, M. N., Bergantz, G. W. (2012). Geological, petrological and geochemical evidence for progressive construction of an arc crustal section, Sierra de Valle Fertil, Famatinian Arc, Argentina. Journal of Petrology 53, 761-800.
Otamendi, J. E., Ducea, M. N., Tibaldi, A. M., Bergantz, G. W., de la Rosa, J. D., Vujovich, G. I. (2009). Generation of tonalitic and dioritic magmas by coupled partial melting of gabbroic and metasedimentary rocks within the deep crust of the Famatinian magmatic arc, Argentina. Journal of Petrology 50, 841-873.
Penniston-Dorland, S. C., Sorensen, S. S., Ash, R. D., Khadke, S. V. (2010). Lithium isotopes as a tracer of fluids in a subduction zone mélange: Franciscan Complex, CA. Earth and Planetary Science Letters 292, 181-190.
Penniston-Dorland, S. C., Walker, R. J., Pitcher, L., Sorensen, S. S. (2012a) Mantle-crust interactions in a paleosubduction zone: Evidence from highly siderophile element systematics of eclogite and related rocks. Earth and Planetary Science Letters. 319-320, 295-306.
Penniston-Dorland, S. C., Bebout, G. E., Pogge von Strandmann, P. A. E., Elliott, T., Sorensen, S. S. (2012b) Lithium and its isotopes as tracers of subduction zone fluids and metasomatic processes: Evidence from the Catalina Schist, California, USA. Geochimica et Cosmochimica Acta. 77, 530-545.
Philippot, P., Selverstone, J. (1991) Trace-element-rich brines in eclogitic veins: implications for fluid composition and transport during subduction. Contributions to Mineralogy and Petrology 106, 417-430.
Pickett, D. A., Saleeby, J. B. (1993). Thermobarometric constraints on the depth of exposure and conditions of plutonism and metamorphism at deep levels of the Sierra Nevada Batholith, Tehachapi Mountains, California. Journal of Geophysical Research 98, 609-629.
Pollington, A.D., Baxter, E.F., (2010) High resolution Sm-Nd garnet geochronology reveals the uneven pace of tectonometamorphic processes. Earth and Planetary Science Letters 293, 63-71.
Pollington, A.D., Baxter, E.F. (2011) High-precision microsampling and preparation of zoned garnet porphyroblasts for Sm/Nd geochronology. Chemical Geology 281, 270-282.
Quick, J. E. (1981). The origin and significance of large, tabular dunite bodies in the Trinity Peridotite, Northern California. Contributions to Mineralogy and Petrology 78, 413-422.
Rapp, R. P., Shimizu, N., Norman, M. D. (2003). Growth of early continental crust by partial melting of eclogite. Nature 425, 605-609.
Reagan, M. K., Ishizuka, O., Stern, R. J., Kelley, K. A., Ohara, Y., Blichert-Toft, J., et al. (2010). Fore-arc basalts and subduction initiation in the Izu-Bonin-Mariana system. Geochemistry, Geophysics, Geosystems 11, Q03X12, doi:10.1029/2009GC002871.
Ringwood, A. E., Green, D. H. (1966). An experimental investigation of the gabbro-eclogite transformation and some geophysical implications. Tectonophysics 3, 383-427.
Rioux, M., Mattinson, J., Hacker, B., Kelemen, P., Blusztajn, J., Hanghoej, K., Gehrels, G. (2010). Intermediate to felsic middle crust in the accreted Talkeetna Arc, the Alaska Peninsula and Kodiak Island, Alaska: An analogue for low-velocity middle crust in modern arcs. Tectonics 29, TC3001, doi:10.1029/2009TC002541.
Rodriguez-Vargas, A., Koester, E., Mallmann, G., Concelcao, R. V., Kawashita, K., Weber, M. B. I. (2005). Mantle diversity beneath the Colombian Andes, Northern volcanic zone: constraints from Sr and Nd isotopes. Lithos 82, 471-484.
Rudnick, R. L. (1995). Making continental crust. Nature 378, 571-578.
Sadofsky, S. J., Bebout, G. E. (2004). Field and isotopic evidence for fluid mobility in the Franciscan Complex: forearc paleohydrogeology to depths of 30 kilometers, International Geology Reviews 46, 1053-1088.
Saleeby, J., Ducea, M., Clemens-Knott, D. (2003). Production and loss of high-density batholithic root, southern Sierra Nevada, California. Tectonics 22, doi:10.1029/2002TC001374.
Scambelluri, M., Philippot, P. (2001) Deep fluids in subduction zones. Lithos 55, 213-227.
Schneider, M. E., Eggler, D. H. (1986). Fluids in equilibrium with peridotite minerals; implications for mantle metasomatism. Geochimica et Cosmochimica Acta 50, 711-724.
Shervais, J. W. (2001). Birth, death, and resurrection; the life cycle of suprasubduction zone ophiolites. Geochemistry, Geophysics, Geosystems 2, doi:10.1029/2000GC000080.
Shervais, J. W., Jean, M. M. (2012) Inside the subduction factory: Modeling fluid mobile element enrichment in the mantle wedge above a subduction zone. Geochimica et Cosmochimica Acta 95, 270-285.
Sinton J .M., Detrick, R. S. (1992). Mid-Ocean Ridge Magma Chambers. Journal of Geophysical Research 97, 197-216.
Sorensen, S. S., Barton, M. D. (1987). Metasomatism and partial melting in a subduction complex; Catalina Schist, Southern California. Geology 15, 115-118.
Sorensen, S.S., Grossman, J.N. (1989). Enrichment of trace elements in garnet amphibolites from a paleo-subduction zone: Catalina Schist, southern California. Geochimica et Cosmochimica Acta. 53, 3155-3177.
Spandler, C., Hermann, J., Faure, K., Mavrogenes, J. A., Arculus, R. J. (2008). The importance of talc and chlorite “hybrid” rocks for volatile recycling through subduction zones; evidence from the high-pressure subduction mélange of New Caledonia. Contributions to Mineralogy and Petrology 155, 181-198.
Spencer, K.J., Hacker, B.R., Kylander-Clark, A.R.C., Andersen, T.B., Cottle, J.M., Stearns, M.A, Poletti, J.E., Seward, G.G.E. (2013). Campaign-style titanite U-Pb dating by laser-ablation ICP: Implications for crustal flow, phase transformations and titanite closure. Chemical Geology 341, 84-101.
Spiegelman, M., Kelemen, P. B. (2003). Extreme chemical variability as a consequence of channelized melt transport. Geochemistry, Geophysics, Geosystems 4, doi:10.1029/2002GC000336.
Stern, R. J., Bloomer, S. H. (1992). Subduction zone infancy; examples from the Eocene Izu-Bonin-Mariana and Jurassic California arcs. Geological Society of America Bulletin 104, 1621-1636.
Stolper, E., Newman, S. (1994). The role of water in the petrogenesis of Mariana Trough magmas. Earth and Planetary Science Letters 121, 293-325.
Straub, S. M., Layne, G. D. (2002). The systematics of boron isotopes in Izu arc front volcanic rocks. Earth and Planetary Science Letters 198, 25-39.
Takahashi, N., Suyehiro, K., Shinohara, M. (1998). Implications from the seismic crustal structure of the northern Izu-Bonin Arc. Island Arc 7, 383-394.
Takahashi, N., Kodaira, S., Klemperer, S. L., Tatsumi, Y., Kaneda, Y., Suyehiro, K. (2007). Crustal structure and evolution of the Mariana intra-oceanic island arc. Geology 35, 203-206.
Tatham, D. J., Lloyd, G. E., Butler, R. W. H., Casey, M. (2008) Amphibole and lower crustal seismic properties. Earth and Planetary Science Letters 267, 118-128.
Turner, S., Hawkesworth, C., Rogers, N., Bartlett, J., Worthington, T., Hergt, J., Pearce, J., Smith, I. (1997) U-238-Th-230 disequilibria, magma petrogenesis, and flux rates beneath the depleted Tonga-Kermadec island arc. Geochimica et Cosmochimica Acta 61, 4855-4884.
Turner, S., Evans, P., Hawkesworth, C. (2001). Ultrafast source-to-surface movement of melt at island arcs from 226Ra- 230Th systematics. Science 292, 1363-1366.
van Keken, P. E., Hacker, B. R., Syracuse, E. M., Abers, G. A. (2011). Subduction factory: 4. Depth‐dependent flux of H2O from subducting slabs worldwide, Journal of Geophysical Research 116, B01401, doi:10.1029/2010JB007922.
Vantongeren J. A., Mathez E. A., Kelemen P. B. (2010). A felsic end to Bushveld differentiation. Journal of Petrology 51, 1891-1912.
Wada, I., Wang, K. (2009). Common depth of slab-mantle decoupling; reconciling diversity and uniformity of subduction zones. Geochemistry, Geophysics, Geosystems 10, Q10009, doi:10.1029/2009GC002570.
Wakabayashi, J. (2012) Subducted sedimentary serpentinite mélanges: Record of multiple burial-exhumation cycles and subduction erosion. Tectonophysics, doi: 10.1016/j.tecto.2011.11.006
Whitney, D. L. (1992). High pressure metamorphism in the western Cordillera of North America: An example from the Skagit Gneiss, North Cascades, Washington. Journal of Metamorphic Geology 10, 71-85.
Whitney, D. L., Miller, R. B., Paterson, S. R. (1999). P-T-t evidence for mechanisms of vertical tectonic motion in a contractional orogen: north-western US and Canadian Cordillera. Journal of Metamorphic Geology 17, 75-90.
Whitney, D. L., Teyssier, C., Rey, P. F. (2009). The consequences of crustal melting in continental subduction. Lithosphere 1, 323-327.
Willner, A. P., Krohe, A., Maresch, W. V. (2000). Interrelated P-T-t-d paths in the Variscan Erzgebirge Dome (Saxony, Germany); constraints on the rapid exhumation of high-pressure rocks from the root zone of a collisional orogen. International Geology Review 42, 64-85.
Willner, A. P., Sebazungu, E., Gerya, T. V., Maresch, W. V., Krohe, A. (2002). Numerical modeling of PT-paths related to rapid exhumation of high-pressure rocks from the crustal root in the Variscan Erzgebirge Dome (Saxony / Germany). Journal of Geodynamics 33, 281-314.
Yokoyama, T., Kobayashi, K., Kuritani, T., Nakamura, E. (2003). Mantle metasomatism and rapid ascent of slab components beneath island arcs; evidence from 238U–230Th–226Ra disequilibria of Miyakejima Volcano, Izu Arc, Japan. Journal of Geophysical Research, 108, 2329, doi:10.1029/2002JB002103.
Zack, T., Stockli, D.F., Luvizotto, G.L., Barth, M.G., Belousova, E., Wolfe, M.R., Hinton, R.W. (2011). In situ U-Pb rutile dating by LA-ICP-MS: 208Pb correction and prospects for geological applications. Contributions to Mineralogy and Petrology 162, 515-530.
Zandt, G., Gilbert, H., Owens, T., Ducea, M. N., Saleeby, J., Jones, C. (2004). Active foundering of a continental arc root beneath the Sierra Nevada, California. Nature 431, 41-46.
Zegers, T. E., van Keken, P. (2001). Middle Archean continent formation by crustal delamination. Geology 29, 1083-1086.